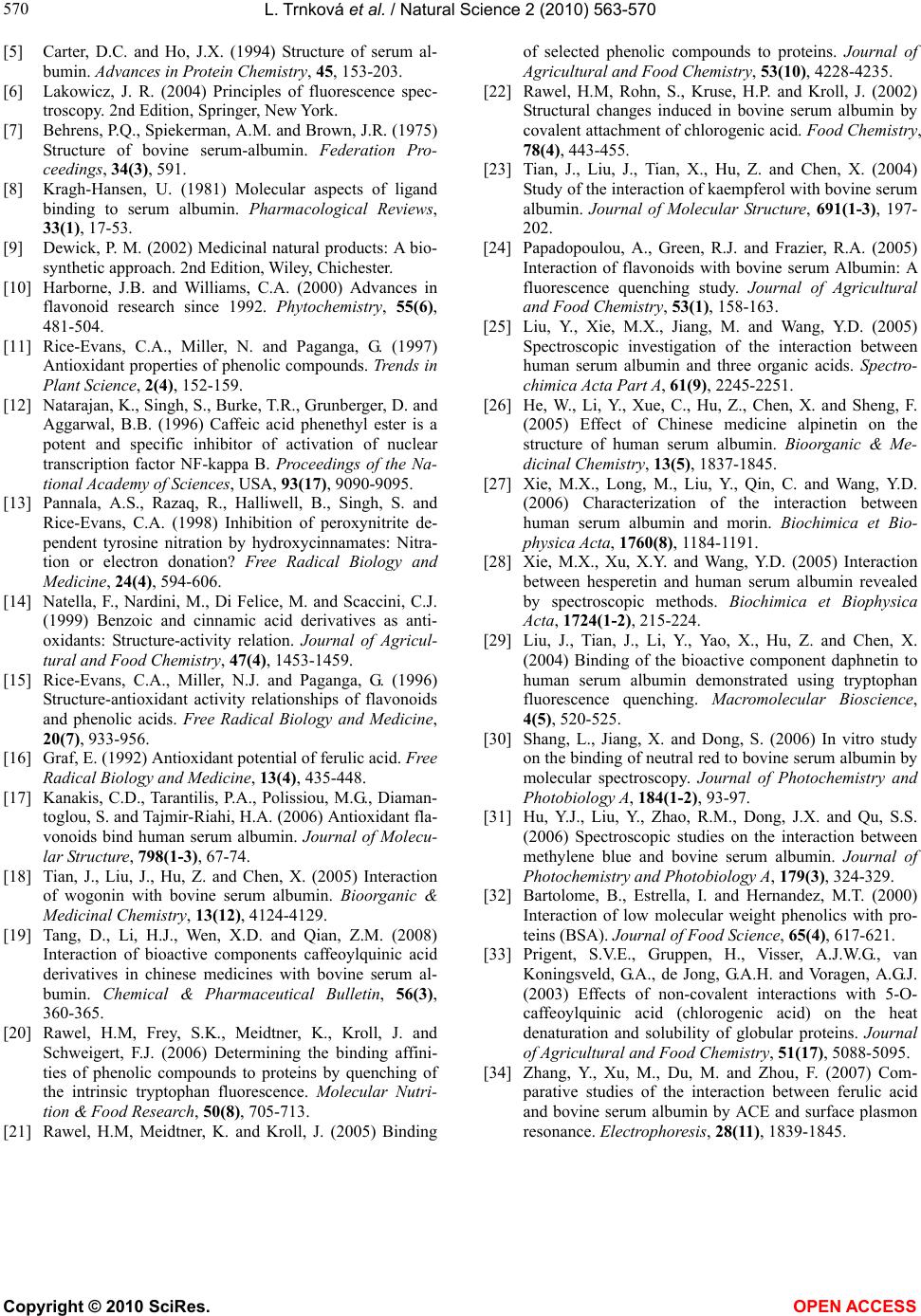
L. Trnková et al. / Natural Science 2 (2010) 563-570
Copyright © 2010 SciRes. OPEN ACCESS
570
[5] Carter, D.C. and Ho, J.X. (1994) Structure of serum al-
bumin. Advances in Protein Chemistry, 45, 153-203.
[6] Lakowicz, J. R. (2004) Principles of fluorescence spec-
troscopy. 2nd Edition, Springer, New York.
[7] Behrens, P.Q., Spiekerman, A.M. and Brown, J.R. (1975)
Structure of bovine serum-albumin. Federation Pro-
ceedings, 34(3), 591.
[8] Kragh-Hansen, U. (1981) Molecular aspects of ligand
binding to serum albumin. Pharmacological Reviews,
33(1), 17-53.
[9] Dewick, P. M. (2002) Medicinal natural products: A bio-
synthetic approach. 2nd Edition, Wiley, Chichester.
[10] Harborne, J.B. and Williams, C.A. (2000) Advances in
flavonoid research since 1992. Phytochemistry, 55(6),
481-504.
[11] Rice-Evans, C.A., Miller, N. and Paganga, G. (1997)
Antioxidant properties of phenolic compounds. Trends in
Plant Science, 2(4), 152-159.
[12] Natarajan, K., Singh, S., Burke, T.R., Grunberger, D. and
Aggarwal, B.B. (1996) Caffeic acid phenethyl ester is a
potent and specific inhibitor of activation of nuclear
transcription factor NF-kappa B. Proceedings of the Na-
tional Academy of Sciences, USA, 93(17), 9090-9095.
[13] Pannala, A.S., Razaq, R., Halliwell, B., Singh, S. and
Rice-Evans, C.A. (1998) Inhibition of peroxynitrite de-
pendent tyrosine nitration by hydroxycinnamates: Nitra-
tion or electron donation? Free Radical Biology and
Medicine, 24(4), 594-606.
[14] Natella, F., Nardini, M., Di Felice, M. and Scaccini, C.J.
(1999) Benzoic and cinnamic acid derivatives as anti-
oxidants: Structure-activity relation. Journal of Agricul-
tural and Food Chemistry, 47(4), 1453-1459.
[15] Rice-Evans, C.A., Miller, N.J. and Paganga, G. (1996)
Structure-antioxidant activity relationships of flavonoids
and phenolic acids. Free Radical Biology and Medicine,
20(7), 933-956.
[16] Graf, E. (1992) Antioxidant potential of ferulic acid. Free
Radical Biology and Medicine, 13(4) , 435-448.
[17] Kanakis, C.D., Tarantilis, P.A., Polissiou, M.G., Diaman-
toglou, S. and Tajmir-Riahi, H.A. (2006) Antioxidant fla-
vonoids bind human serum albumin. Journal of Molecu-
lar Structure, 798(1-3), 67-74.
[18] Tian, J., Liu, J., Hu, Z. and Chen, X. (2005) Interaction
of wogonin with bovine serum albumin. Bioorganic &
Medicinal Chemistry, 13(12), 4124-4129.
[19] Tang, D., Li, H.J., Wen, X.D. and Qian, Z.M. (2008)
Interaction of bioactive components caffeoylquinic acid
derivatives in chinese medicines with bovine serum al-
bumin. Chemical & Pharmaceutical Bulletin, 56(3),
360-365.
[20] Rawel, H.M, Frey, S.K., Meidtner, K., Kroll, J. and
Schweigert, F.J. (2006) Determining the binding affini-
ties of phenolic compounds to proteins by quenching of
the intrinsic tryptophan fluorescence. Molecular Nutri-
tion & Food Research, 50(8), 705-713.
[21] Rawel, H.M, Meidtner, K. and Kroll, J. (2005) Binding
of selected phenolic compounds to proteins. Journal of
Agricultural and Food Chemistry, 53(10), 4228-4235.
[22] Rawel, H.M, Rohn, S., Kruse, H.P. and Kroll, J. (2002)
Structural changes induced in bovine serum albumin by
covalent attachment of chlorogenic acid. Food Chemistry,
78(4), 443-455.
[23] Tian, J., Liu, J., Tian, X., Hu, Z. and Chen, X. (2004)
Study of the interaction of kaempferol with bovine serum
albumin. Journal of Molecular Structure, 691(1-3), 197-
202.
[24] Papadopoulou, A., Green, R.J. and Frazier, R.A. (2005)
Interaction of flavonoids with bovine serum Albumin: A
fluorescence quenching study. Journal of Agricultural
and Food Chemistry, 53(1), 158-163.
[25] Liu, Y., Xie, M.X., Jiang, M. and Wang, Y.D. (2005)
Spectroscopic investigation of the interaction between
human serum albumin and three organic acids. Spectro-
chimica Acta Part A, 61(9), 2245-2251.
[26] He, W., Li, Y., Xue, C., Hu, Z., Chen, X. and Sheng, F.
(2005) Effect of Chinese medicine alpinetin on the
structure of human serum albumin. Bioorganic & Me-
dicinal Chemistry, 13(5), 1837-1845.
[27] Xie, M.X., Long, M., Liu, Y., Qin, C. and Wang, Y.D.
(2006) Characterization of the interaction between
human serum albumin and morin. Biochimica et Bio-
physica Acta, 1760(8), 1184-1191.
[28] Xie, M.X., Xu, X.Y. and Wang, Y.D. (2005) Interaction
between hesperetin and human serum albumin revealed
by spectroscopic methods. Biochimica et Biophysica
Acta, 1724(1-2), 215-224.
[29] Liu, J., Tian, J., Li, Y., Yao, X., Hu, Z. and Chen, X.
(2004) Binding of the bioactive component daphnetin to
human serum albumin demonstrated using tryptophan
fluorescence quenching. Macromolecular Bioscience,
4(5), 520-525.
[30] Shang, L., Jiang, X. and Dong, S. (2006) In vitro study
on the binding of neutral red to bovine serum albumin by
molecular spectroscopy. Journal of Photochemistry and
Photobiology A, 184(1-2), 93-97.
[31] Hu, Y.J., Liu, Y., Zhao, R.M., Dong, J.X. and Qu, S.S.
(2006) Spectroscopic studies on the interaction between
methylene blue and bovine serum albumin. Journal of
Photochemistry and Photobiology A, 179(3), 324-329.
[32] Bartolome, B., Estrella, I. and Hernandez, M.T. (2000)
Interaction of low molecular weight phenolics with pro-
teins (BSA). Journal of Food Science, 65(4), 617-621.
[33] Prigent, S.V.E., Gruppen, H., Visser, A.J.W.G., van
Koningsveld, G.A., de Jong, G.A.H. and Voragen, A.G.J.
(2003) Effects of non-covalent interactions with 5-O-
caffeoylquinic acid (chlorogenic acid) on the heat
denaturation and solubility of globular proteins. Journal
of Agricultural and Food Chemistry, 51(17), 5088-5095.
[34] Zhang, Y., Xu, M., Du, M. and Zhou, F. (2007) Com-
parative studies of the interaction between ferulic acid
and bovine serum albumin by ACE and surface plasmon
resonance. Electrophoresis, 28(11), 1839-1845.