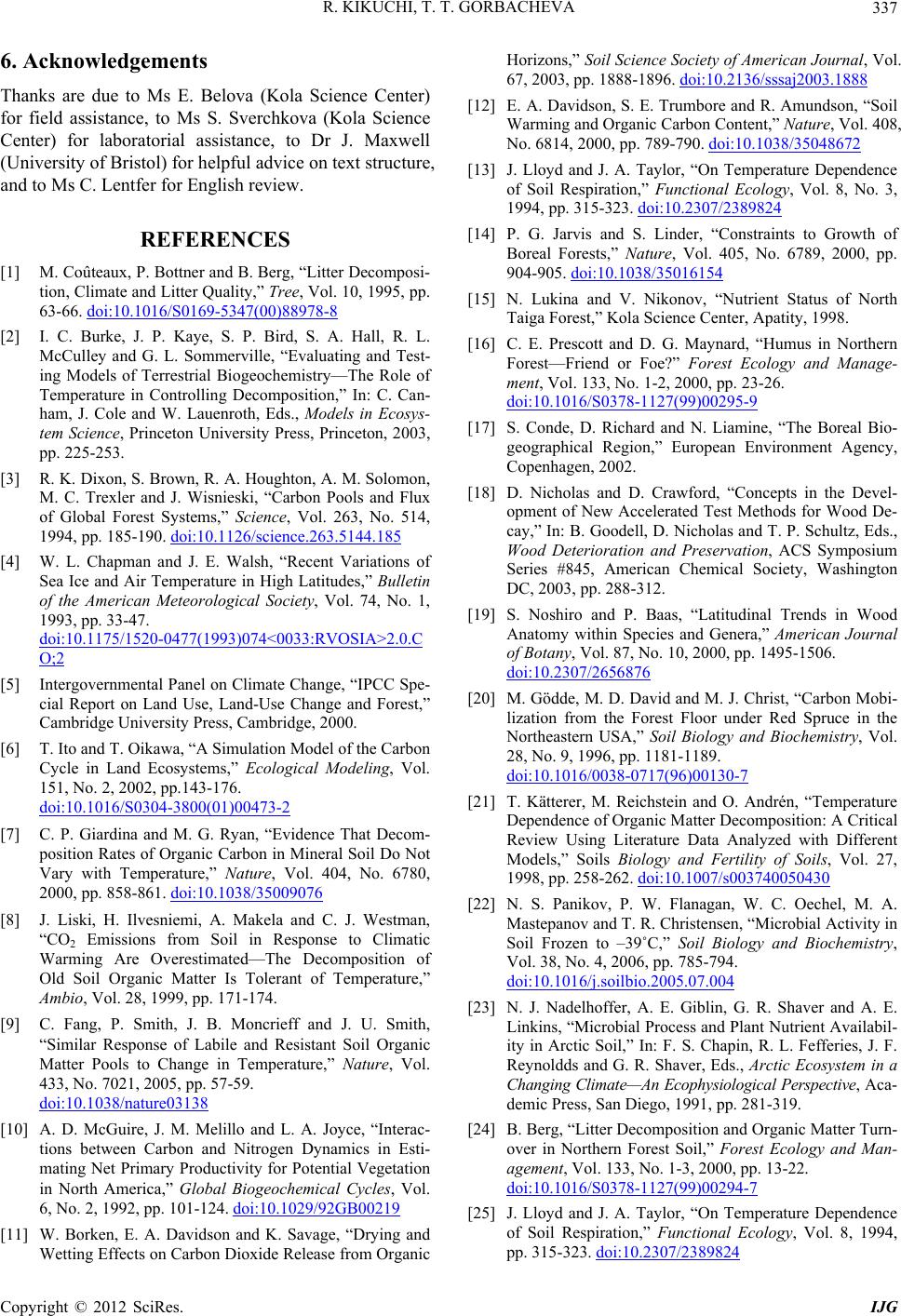
R. KIKUCHI, T. T. GORBACHEVA 337
6. Acknowledgements
Thanks are due to Ms E. Belova (Kola Science Center)
for field assistance, to Ms S. Sverchkova (Kola Science
Center) for laboratorial assistance, to Dr J. Maxwell
(University of Bristol) for helpful advice on text structure,
and to Ms C. Lentfer for English review.
REFERENCES
[1] M. Coûteaux, P. Bottner and B. Berg, “Litter Decomposi-
tion, Climate and Litter Quality,” Tree, Vol. 10, 1995, pp.
63-66. doi:10.1016/S0169-5347(00)88978-8
[2] I. C. Burke, J. P. Kaye, S. P. Bird, S. A. Hall, R. L.
McCulley and G. L. Sommerville, “Evaluating and Test-
ing Models of Terrestrial Biogeochemistry—The Role of
Temperature in Controlling Decomposition,” In: C. Can-
ham, J. Cole and W. Lauenroth, Eds., Models in Ecosys-
tem Science, Princeton University Press, Princeton, 2003,
pp. 225-253.
[3] R. K. Dixon, S. Brown, R. A. Houghton, A. M. Solomon,
M. C. Trexler and J. Wisnieski, “Carbon Pools and Flux
of Global Forest Systems,” Science, Vol. 263, No. 514,
1994, pp. 185-190. doi:10.1126/science.263.5144.185
[4] W. L. Chapman and J. E. Walsh, “Recent Variations of
Sea Ice and Air Temperature in High Latitudes,” Bulletin
of the American Meteorological Society, Vol. 74, No. 1,
1993, pp. 33-47.
doi:10.1175/1520-0477(1993)074<0033:RVOSIA>2.0.C
O;2
[5] Intergovernmental Panel on Climate Change, “IPCC Spe-
cial Report on Land Use, Land-Use Change and Forest,”
Cambridge University Press, Cambridge, 2000.
[6] T. Ito and T. Oikawa, “A Simulation Model of the Carbon
Cycle in Land Ecosystems,” Ecological Modeling, Vol.
151, No. 2, 2002, pp.143-176.
doi:10.1016/S0304-3800(01)00473-2
[7] C. P. Giardina and M. G. Ryan, “Evidence That Decom-
position Rates of Organic Carbon in Mineral Soil Do Not
Vary with Temperature,” Nature, Vol. 404, No. 6780,
2000, pp. 858-861. doi:10.1038/35009076
[8] J. Liski, H. Ilvesniemi, A. Makela and C. J. Westman,
“CO2 Emissions from Soil in Response to Climatic
Warming Are Overestimated—The Decomposition of
Old Soil Organic Matter Is Tolerant of Temperature,”
Ambio, Vol. 28, 1999, pp. 171-174.
[9] C. Fang, P. Smith, J. B. Moncrieff and J. U. Smith,
“Similar Response of Labile and Resistant Soil Organic
Matter Pools to Change in Temperature,” Nature, Vol.
433, No. 7021, 2005, pp. 57-59.
doi:10.1038/nature03138
[10] A. D. McGuire, J. M. Melillo and L. A. Joyce, “Interac-
tions between Carbon and Nitrogen Dynamics in Esti-
mating Net Primary Productivity for Potential Vegetation
in North America,” Global Biogeochemical Cycles, Vol.
6, No. 2, 1992, pp. 101-124. doi:10.1029/92GB00219
[11] W. Borken, E. A. Davidson and K. Savage, “Drying and
Wetting Effects on Carbon Dioxide Release from Organic
Horizons,” Soil Science Society of American Journal, Vol.
67, 2003, pp. 1888-1896. doi:10.2136/sssaj2003.1888
[12] E. A. Davidson, S. E. Trumbore and R. Amundson, “Soil
Warming and Organic Carbon Content,” Nature, Vol. 408,
No. 6814, 2000, pp. 789-790. doi:10.1038/35048672
[13] J. Lloyd and J. A. Taylor, “On Temperature Dependence
of Soil Respiration,” Functional Ecology, Vol. 8, No. 3,
1994, pp. 315-323. doi:10.2307/2389824
[14] P. G. Jarvis and S. Linder, “Constraints to Growth of
Boreal Forests,” Nature, Vol. 405, No. 6789, 2000, pp.
904-905. doi:10.1038/35016154
[15] N. Lukina and V. Nikonov, “Nutrient Status of North
Taiga Forest,” Kola Science Center, Apatity, 1998.
[16] C. E. Prescott and D. G. Maynard, “Humus in Northern
Forest—Friend or Foe?” Forest Ecology and Manage-
ment, Vol. 133, No. 1-2, 2000, pp. 23-26.
doi:10.1016/S0378-1127(99)00295-9
[17] S. Conde, D. Richard and N. Liamine, “The Boreal Bio-
geographical Region,” European Environment Agency,
Copenhagen, 2002.
[18] D. Nicholas and D. Crawford, “Concepts in the Devel-
opment of New Accelerated Test Methods for Wood De-
cay,” In: B. Goodell, D. Nicholas and T. P. Schultz, Eds.,
Wood Deterioration and Preservation, ACS Symposium
Series #845, American Chemical Society, Washington
DC, 2003, pp. 288-312.
[19] S. Noshiro and P. Baas, “Latitudinal Trends in Wood
Anatomy within Species and Genera,” American Journal
of Botany, Vol. 87, No. 10, 2000, pp. 1495-1506.
doi:10.2307/2656876
[20] M. Gödde, M. D. David and M. J. Christ, “Carbon Mobi-
lization from the Forest Floor under Red Spruce in the
Northeastern USA,” Soil Biology and Biochemistry, Vol.
28, No. 9, 1996, pp. 1181-1189.
doi:10.1016/0038-0717(96)00130-7
[21] T. Kätterer, M. Reichstein and O. Andrén, “Temperature
Dependence of Organic Matter Decomposition: A Critical
Review Using Literature Data Analyzed with Different
Models,” Soils Biology and Fertility of Soils, Vol. 27,
1998, pp. 258-262. doi:10.1007/s003740050430
[22] N. S. Panikov, P. W. Flanagan, W. C. Oechel, M. A.
Mastepanov and T. R. Christensen, “Microbial Activity in
Soil Frozen to –39˚C,” Soil Biology and Biochemistry,
Vol. 38, No. 4, 2006, pp. 785-794.
doi:10.1016/j.soilbio.2005.07.004
[23] N. J. Nadelhoffer, A. E. Giblin, G. R. Shaver and A. E.
Linkins, “Microbial Process and Plant Nutrient Availabil-
ity in Arctic Soil,” In: F. S. Chapin, R. L. Fefferies, J. F.
Reynoldds and G. R. Shaver, Eds., Arctic Ecosystem in a
Changing Climat e—An Ecophysiological Pe rspective, Aca-
demic Press, San Diego, 1991, pp. 281-319.
[24] B. Berg, “Litter Decomposition and Organic Matter Turn-
over in Northern Forest Soil,” Forest Ecology and Man-
agement, Vol. 133, No. 1-3, 2000, pp. 13-22.
doi:10.1016/S0378-1127(99)00294-7
[25] J. Lloyd and J. A. Taylor, “On Temperature Dependence
of Soil Respiration,” Functional Ecology, Vol. 8, 1994,
pp. 315-323. doi:10.2307/2389824
Copyright © 2012 SciRes. IJG