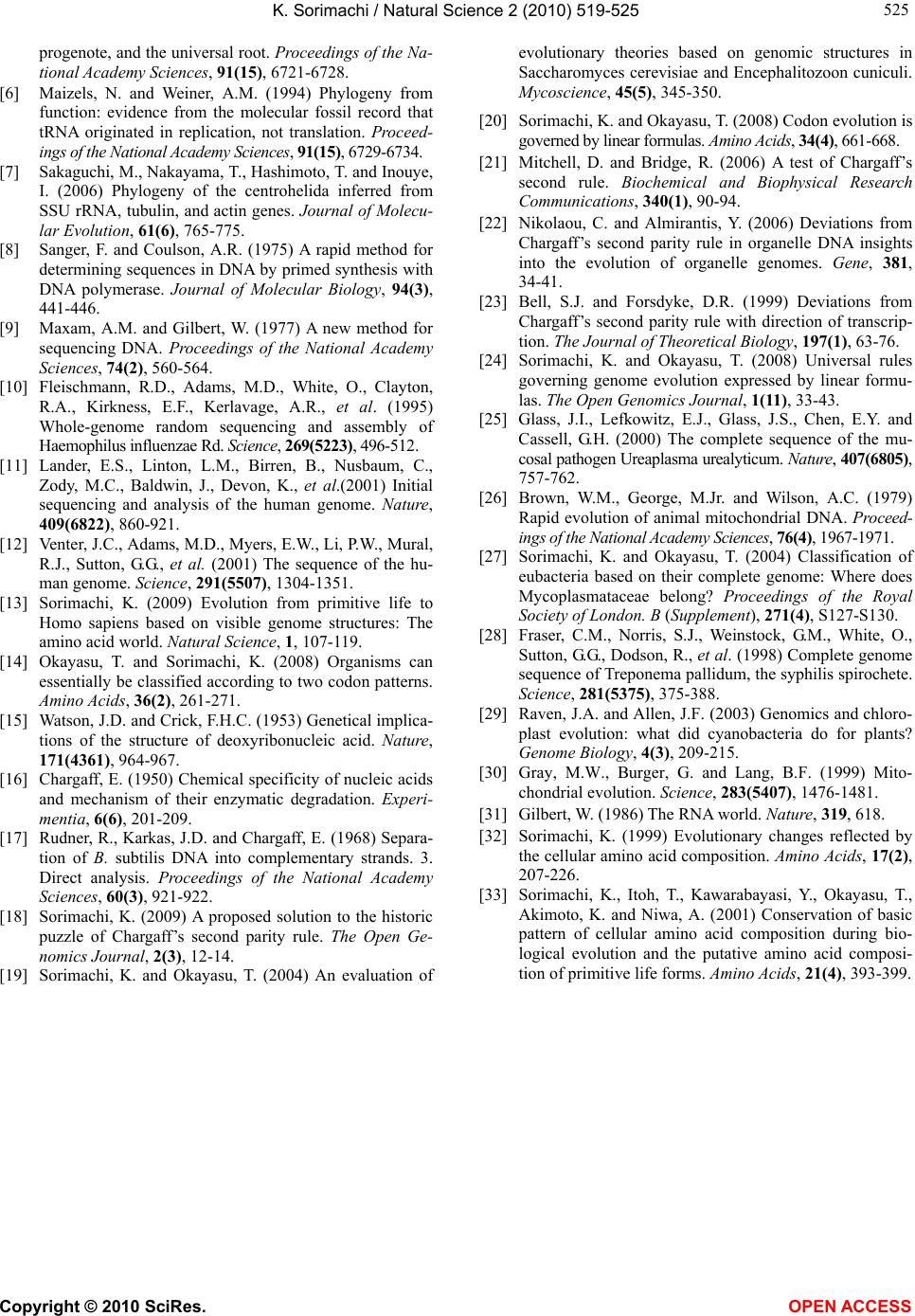
K. Sorimachi / Natural Science 2 (2010) 519-525
Copyright © 2010 SciRes. OPEN ACCESS
525
525
progenote, and the universal root. Proceedings of the Na-
tional Academy Sciences, 91(15), 6721-6728.
[6] Maizels, N. and Weiner, A.M. (1994) Phylogeny from
function: evidence from the molecular fossil record that
tRNA originated in replication, not translation. Proceed-
ings of the National Academy Sciences, 91(15), 6729-6734.
[7] Sakaguchi, M., Nakayama, T., Hashimoto, T. and Inouye,
I. (2006) Phylogeny of the centrohelida inferred from
SSU rRNA, tubulin, and actin genes. Journal of Molecu-
lar Evolution, 61(6), 765-775.
[8] Sanger, F. and Coulson, A.R. (1975) A rapid method for
determining sequences in DNA by primed synthesis with
DNA polymerase. Journal of Molecular Biology, 94(3),
441-446.
[9] Maxam, A.M. and Gilbert, W. (1977) A new method for
sequencing DNA. Proceedings of the National Academy
Sciences, 74(2), 560-564.
[10] Fleischmann, R.D., Adams, M.D., White, O., Clayton,
R.A., Kirkness, E.F., Kerlavage, A.R., et al. (1995)
Whole-genome random sequencing and assembly of
Haemophilus influenzae Rd. Science, 269(5223), 496-512.
[11] Lander, E.S., Linton, L.M., Birren, B., Nusbaum, C.,
Zody, M.C., Baldwin, J., Devon, K., et al.(2001) Initial
sequencing and analysis of the human genome. Nature,
409(6822), 860-921.
[12] Venter, J.C., Adams, M.D., Myers, E.W., Li, P.W., Mural,
R.J., Sutton, G.G., et al. (2001) The sequence of the hu-
man genome. Science, 291(5507), 1304-1351.
[13] Sorimachi, K. (2009) Evolution from primitive life to
Homo sapiens based on visible genome structures: The
amino acid world. Natural Science, 1, 107-119.
[14] Okayasu, T. and Sorimachi, K. (2008) Organisms can
essentially be classified according to two codon patterns.
Amino Acids, 36(2), 261-271.
[15] Watson, J.D. and Crick, F.H.C. (1953) Genetical implica-
tions of the structure of deoxyribonucleic acid. Nature,
171(4361), 964-967.
[16] Chargaff, E. (1950) Chemical specificity of nucleic acids
and mechanism of their enzymatic degradation. Experi-
mentia, 6(6), 201-209.
[17] Rudner, R., Karkas, J.D. and Chargaff, E. (1968) Separa-
tion of B. subtilis DNA into complementary strands. 3.
Direct analysis. Proceedings of the National Academy
Sciences, 60(3), 921-922.
[18] Sorimachi, K. (2009) A proposed solution to the historic
puzzle of Chargaff’s second parity rule. The Open Ge-
nomics Journal, 2(3), 12-14.
[19] Sorimachi, K. and Okayasu, T. (2004) An evaluation of
evolutionary theories based on genomic structures in
Saccharomyces cerevisiae and Encephalitozoon cuniculi.
Mycoscience, 45(5), 345-350.
[20] Sorimachi, K. and Okayasu, T. (2008) Codon evolution is
governed by linear formulas. Amino Acids, 34(4), 661-668.
[21] Mitchell, D. and Bridge, R. (2006) A test of Chargaff’s
second rule. Biochemical and Biophysical Research
Communications, 340(1), 90-94.
[22] Nikolaou, C. and Almirantis, Y. (2006) Deviations from
Chargaff’s second parity rule in organelle DNA insights
into the evolution of organelle genomes. Gene, 381,
34-41.
[23] Bell, S.J. and Forsdyke, D.R. (1999) Deviations from
Chargaff’s second parity rule with direction of transcrip-
tion. The Journal of Theoretical Biology, 197(1), 63-76.
[24] Sorimachi, K. and Okayasu, T. (2008) Universal rules
governing genome evolution expressed by linear formu-
las. The Open Genomics Journal, 1(11), 33-43.
[25] Glass, J.I., Lefkowitz, E.J., Glass, J.S., Chen, E.Y. and
Cassell, G
.H. (2000) The complete sequence of the mu-
cosal pathogen Ureaplasma urealyticum. Nature, 407(6805),
757-762.
[26] Brown, W.M., George, M.Jr. and Wilson, A.C. (1979)
Rapid evolution of animal mitochondrial DNA. Proceed-
ings of the National Academy Sciences, 76(4), 1967-1971.
[27] Sorimachi, K. and Okayasu, T. (2004) Classification of
eubacteria based on their complete genome: Where does
Mycoplasmataceae belong? Proceedings of the Royal
Society of London. B (Supplement), 271(4), S127-S130.
[28] Fraser, C.M., Norris, S.J., Weinstock, G.M., White, O.,
Sutton, G.G., Dodson, R., et al. (1998) Complete genome
sequence of Treponema pallidum, the syphilis spirochete.
Science, 281(5375), 375-388.
[29] Raven, J.A. and Allen, J.F. (2003) Genomics and chloro-
plast evolution: what did cyanobacteria do for plants?
Genome Biology, 4(3), 209-215.
[30] Gray, M.W., Burger, G. and Lang, B.F. (1999) Mito-
chondrial evolution. Science, 283(5407), 1476-1481.
[31] Gilbert, W. (1986) The RNA world. Nature, 319, 618.
[32] Sorimachi, K. (1999) Evolutionary changes reflected by
the cellular amino acid composition. Amino Acids, 17(2),
207-226.
[33] Sorimachi, K., Itoh, T., Kawarabayasi, Y., Okayasu, T.,
Akimoto, K. and Niwa, A. (2001) Conservation of basic
pattern of cellular amino acid composition during bio-
logical evolution and the putative amino acid composi-
tion of primitive life forms. Amino Acids, 21(4), 393-399.