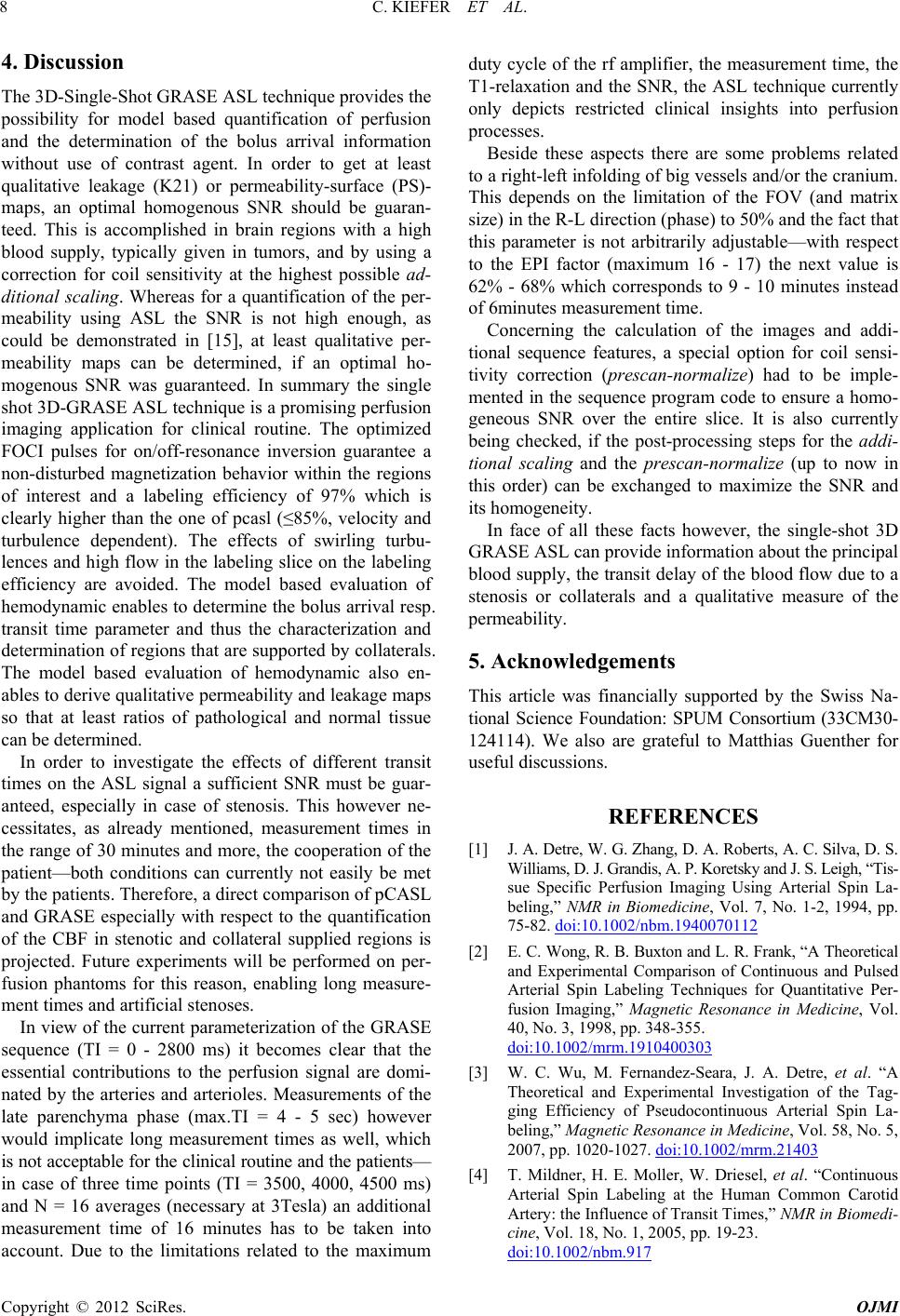
C. KIEFER ET AL.
Copyright © 2012 SciRes. OJMI
8
4. Discussion
The 3D-Single-Shot GRASE ASL technique provides the
possibility for model based quantification of perfusion
and the determination of the bolus arrival information
without use of contrast agent. In order to get at least
qualitative leakage (K21) or permeability-surface (PS)-
maps, an optimal homogenous SNR should be guaran-
teed. This is accomplished in brain regions with a high
blood supply, typically given in tumors, and by using a
correction for coil sensitivity at the highest possible ad-
ditional scaling. Whereas for a quantification of the per-
meability using ASL the SNR is not high enough, as
could be demonstrated in [15], at least qualitative per-
meability maps can be determined, if an optimal ho-
mogenous SNR was guaranteed. In summary the single
shot 3D-GRASE ASL technique is a promising perfusion
imaging application for clinical routine. The optimized
FOCI pulses for on/off-resonance inversion guarantee a
non-disturbed magnetization behavior within the regions
of interest and a labeling efficiency of 97% which is
clearly higher than the one of pcasl (≤85%, velocity and
turbulence dependent). The effects of swirling turbu-
lences and high flow in the labeling slice on the labeling
efficiency are avoided. The model based evaluation of
hemodynamic enables to determine the bolus arrival resp.
transit time parameter and thus the characterization and
determination of regions that are supported by collaterals.
The model based evaluation of hemodynamic also en-
ables to derive qualitative permeability and leakage maps
so that at least ratios of pathological and normal tissue
can be determined.
In order to investigate the effects of different transit
times on the ASL signal a sufficient SNR must be guar-
anteed, especially in case of stenosis. This however ne-
cessitates, as already mentioned, measurement times in
the range of 30 minutes and more, the cooperation of the
patient—both conditions can currently not easily be met
by the patients. Therefore, a direct comparison of pCASL
and GRASE especially with respect to the quantification
of the CBF in stenotic and collateral supplied regions is
projected. Future experiments will be performed on per-
fusion phantoms for this reason, enabling long measure-
ment times and artificial stenoses.
In view of the current parameterization of the GRASE
sequence (TI = 0 - 2800 ms) it becomes clear that the
essential contributions to the perfusion signal are domi-
nated by the arteries and arterioles. Measurements of the
late parenchyma phase (max.TI = 4 - 5 sec) however
would implicate long measurement times as well, which
is not acceptable for the clinical routine and the patients—
in case of three time points (TI = 3500, 4000, 4500 ms)
and N = 16 averages (necessary at 3Tesla) an additional
measurement time of 16 minutes has to be taken into
account. Due to the limitations related to the maximum
duty cycle of the rf amplifier, the measurement time, the
T1-relaxation and the SNR, the ASL technique currently
only depicts restricted clinical insights into perfusion
processes.
Beside these aspects there are some problems related
to a right-left infolding of big vessels and/or the cranium.
This depends on the limitation of the FOV (and matrix
size) in the R-L direction (phase) to 50% and the fact that
this parameter is not arbitrarily adjustable—with respect
to the EPI factor (maximum 16 - 17) the next value is
62% - 68% which corresponds to 9 - 10 minutes instead
of 6minutes measurement time.
Concerning the calculation of the images and addi-
tional sequence features, a special option for coil sensi-
tivity correction (prescan-normalize) had to be imple-
mented in the sequence program code to ensure a homo-
geneous SNR over the entire slice. It is also currently
being checked, if the post-processing steps for the addi-
tional scaling and the prescan-normalize (up to now in
this order) can be exchanged to maximize the SNR and
its homogeneity.
In face of all these facts however, the single-shot 3D
GRASE ASL can provide information about the principal
blood supply, the transit delay of the blood flow due to a
stenosis or collaterals and a qualitative measure of the
permeability.
5. Acknowledgements
This article was financially supported by the Swiss Na-
tional Science Foundation: SPUM Consortium (33CM30-
124114). We also are grateful to Matthias Guenther for
useful discussions.
REFERENCES
[1] J. A. Detre, W. G. Zhang, D. A. Roberts, A. C. Silva, D. S.
Williams, D. J. Grandis, A. P. Koretsky and J. S. Leigh, “Tis-
sue Specific Perfusion Imaging Using Arterial Spin La-
beling,” NMR in Biomedicine, Vol. 7, No. 1-2, 1994, pp.
75-82. doi:10.1002/nbm.1940070112
[2] E. C. Wong, R. B. Buxton and L. R. Frank, “A Theoretical
and Experimental Comparison of Continuous and Pulsed
Arterial Spin Labeling Techniques for Quantitative Per-
fusion Imaging,” Magnetic Resonance in Medicine, Vol.
40, No. 3, 1998, pp. 348-355.
doi:10.1002/mrm.1910400303
[3] W. C. Wu, M. Fernandez-Seara, J. A. Detre, et al. “A
Theoretical and Experimental Investigation of the Tag-
ging Efficiency of Pseudocontinuous Arterial Spin La-
beling,” Magnetic Resonance in Medicine, Vol. 58, No. 5,
2007, pp. 1020-1027. doi:10.1002/mrm.21403
[4] T. Mildner, H. E. Moller, W. Driesel, et al. “Continuous
Arterial Spin Labeling at the Human Common Carotid
Artery: the Influence of Transit Times,” NMR in Bi omedi-
cine, Vol. 18, No. 1, 2005, pp. 19-23.
doi:10.1002/nbm.917