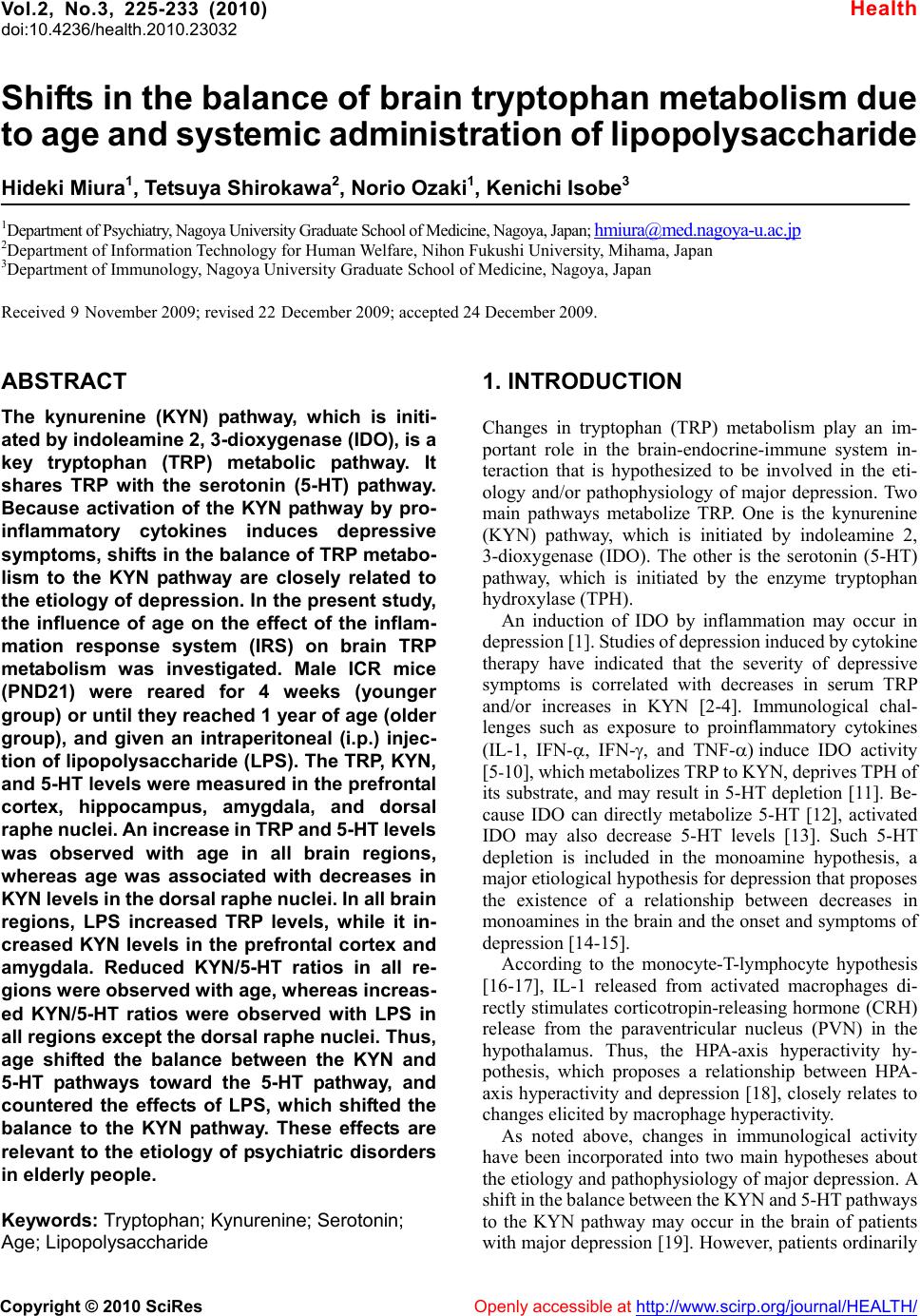 Vol.2, No.3, 225-233 (2010) doi:10.4236/health.2010.23032 Copyright © 2010 SciRes Openly accessible at http://www.scirp.org/journal/HEALTH/ Health Shifts in the balance of brain tryptophan metabolism due to age and systemic administration of lipopolysaccharide Hideki Miura1, Tetsuya Shirokawa2, Norio Ozaki1, Kenichi Isobe3 1Department of Psychiatry, Nagoya University Graduate School of Medicine, Nagoya, Japan; hmiura@med.nagoya-u.ac.jp 2Department of Information Technology for Human Welfare, Nihon Fukushi University, Mihama, Japan 3Department of Immunology, Nagoya University Graduate School of Medicine, Nagoya, Japan Received 9 November 2009; revised 22 December 2009; accepted 24 December 2009. ABSTRACT The kynurenine (KYN) pathway, which is initi- ated by indoleamine 2, 3-dioxygenase (IDO), is a key tryptophan (TRP) metabolic pathway. It shares TRP with the serotonin (5-HT) pathway. Because activation of the KYN pathway by pro- inflammatory cytokines induces depressive symptoms, shifts in the balance of TRP metabo- lism to the KYN pathway are closely related to the etiology of depression. In the present study, the influence of age on the effect of the inflam- mation response system (IRS) on brain TRP metabolism was investigated. Male ICR mice (PND21) were reared for 4 weeks (younger group) or until they reached 1 year of age (older group), and given an intraperitoneal (i.p.) injec- tion of lipopolysaccharide (LPS). The TRP, KYN, and 5-HT levels were measured in the prefrontal cortex, hippocampus, amygdala, and dorsal raphe nuclei. An increase in TRP and 5-HT levels was observed with age in all brain regions, whereas age was associated with decreases in KYN levels in the dorsal raphe nuclei. In all brain regions, LPS increased TRP levels, while it in- creased KYN levels in the prefrontal cortex and amygdala. Reduced KYN/5-HT ratios in all re- gions were observed with age, whereas increas- ed KYN/5-HT ratios were observed with LPS in all regions except the dorsal raphe nuclei. Thus, age shifted the balance between the KYN and 5-HT pathways toward the 5-HT pathway, and countered the effects of LPS, which shifted the balance to the KYN pathway. These effects are relevant to the etiology of psychiatric disorders in elderly people. Keywords: Tryptophan; Kynurenine; Serotonin; Age; Lipopolysaccharide 1. INTRODUCTION Changes in tryptophan (TRP) metabolism play an im- portant role in the brain-endocrine-immune system in- teraction that is hypothesized to be involved in the eti- ology and/or pathophysiology of major depression. Two main pathways metabolize TRP. One is the kynurenine (KYN) pathway, which is initiated by indoleamine 2, 3-dioxygenase (IDO). The other is the serotonin (5-HT) pathway, which is initiated by the enzyme tryptophan hydroxylase (TPH). An induction of IDO by inflammation may occur in depression [1]. Studies of depression induced by cytokine therapy have indicated that the severity of depressive symptoms is correlated with decreases in serum TRP and/or increases in KYN [2-4]. Immunological chal- lenges such as exposure to proinflammatory cytokines (IL-1, IFN-, IFN-, and TNF-induce IDO activity [5-10], which metabolizes TRP to KYN, deprives TPH of its substrate, and may result in 5-HT depletion [11]. Be- cause IDO can directly metabolize 5-HT [12], activated IDO may also decrease 5-HT levels [13]. Such 5-HT depletion is included in the monoamine hypothesis, a major etiological hypothesis for depression that proposes the existence of a relationship between decreases in monoamines in the brain and the onset and symptoms of depression [14-15]. According to the monocyte-T-lymphocyte hypothesis [16-17], IL-1 released from activated macrophages di- rectly stimulates corticotropin-releasing hormone (CRH) release from the paraventricular nucleus (PVN) in the hypothalamus. Thus, the HPA-axis hyperactivity hy- pothesis, which proposes a relationship between HPA- axis hyperactivity and depression [18], closely relates to changes elicited by macrophage hyperactivity. As noted above, changes in immunological activity have been incorporated into two main hypotheses about the etiology and pathophysiology of major depression. A shift in the balance between the KYN and 5-HT pathways to the KYN pathway may occur in the brain of patients with major depression [19]. However, patients ordinarily
 H. Miura et al. / HEALTH 2 (2010) 225-233 Copyright © 2010 SciRes Openly accessible at http://www.scirp.org/journal/HEALTH/ 226 become depressed in response to adverse life events and/or the loss of social support (i.e., psychological and/or environmental factors), especially when such events or losses are encountered at an advanced age [20-21]. In order to investigate the shift in the balance in the TRP metabolism to the KYN pathway, the influence of such factors on TRP metabolism was investigated [22]. Specifically, a previously designed animal model [23-28] was applied to assess the influence of the following three risk factors on TRP metabolism: age, social isolation, and activation of the inflammation response system (IRS). The results showed that older age and social isolation shifted the balance between the KYN and 5-HT pathways to the 5-HT pathway, whereas novelty stress shifted the balance to the KYN pathway [22]. Further study revealed that immunological challenges such as intraperitoneal (i.p.) injection of lipopolysaccharide (LPS) shifted the balance to the KYN pathway [29]. These studies may confirm that psychological stressors, as well as immu- nological challenges, can shift the balance between the KYN and 5-HT pathways toward the KYN pathway, as expected. Although interactions between social isolation and systemic LPS injection on TRP metabolism in the brain have been observed [29], the influence of age on changes elicited by systemic LPS injection remains to be examined. The aim of the present study was to clarify the influ- ence of age on a shift in the balance between the KYN and 5-HT pathways elicited by i.p. injection of LPS. For this series, four brain regions were selected, the first three of which possess 5-HT nerve terminals: the prefrontal cortex, because it relates to behavioral motivation; the amygdala, because it relates to emotion; the hippocampus, because it regulates the HPA axis, and hyperactivity of this axis is closely related to the etiology and patho- physiology of depression; and the dorsal raphe nuclei, because they contain the cell bodies of 5-HT neurons and are the center of brain 5-HT synthesis. 2. METHODOLOGY 2.1. Animals A total of 32 male-specific, pathogen-free (SPF) ICR mice were used in the present experiments. At 21 post- natal days (PND), the mice were housed in groups of 4–5 per cage, and they were reared for 4 weeks (younger group) or until they became 1 year old (older group). On the final day (Day 28 for the younger group, and 1 year old for the older group), the animals were further sepa- rated into two groups and were then given a 0 mg/kg or 1 mg/kg intraperitoneal (i.p.) injection of LPS diluted with saline. Thus, the mice were divided into 4 groups as follows: younger, LPS 0 mg/kg (n=8); younger, LPS 1 mg/kg (n=8); older, LPS 0 mg/kg (n=8); older, LPS 1 mg/kg (n=8). Mice in the older group with apparent wounds were excluded from the study, because any such wound would itself likely have influenced the immune response, and in turn TRP metabolism. Although this selection process excluded apparently subordinate mice from the study, it was more important to avoid the potential influence of the immune responses associated with wounds on TRP metabolism. The cages used in this series measured 21 x 31 x 13 cm. Cage exchange was performed 2 times per week. Food and water were provided ad libitum. The animals were kept on a 12-h light/dark cycle (lights on at 09.00 h, off at 21.00 h), and room temperature was maintained at 21–23˚C. All efforts were made to minimize both the number of animals used and the degree of their suffering. All experiments were conducted in accordance with the European Communities Council Directive of November 24, 1986 (86/609/EEC). The study was approved by the ethical committee of the Nagoya University Graduate School of Medicine. 2.2. LPS Injection For the LPS injection, LPS from E. coli (Sigma, St. Louis, MO) was diluted with saline (2 mg/10 ml) and i.p.-injected (0 mg/kg or 1 mg/kg) into the mice (saline only in the 0 mg/kg group). The time of LPS injection was between 11.00 h and 14.00 h. The injection volume was 5 ml/kg. After having received the LPS injection, the mice were returned to their rearing cages until brain sample preparation. 2.3. Sample Preparation Four brain regions (prefrontal cortex, hippocampus, amygdala, and dorsal raphe nuclei) were dissected from the whole brain. The mice were sacrificed by decapitation 4 h after the i.p.-injection of LPS; mice were decapitated under brief ether anesthesia. The brains were removed, and four brain regions were dissected out as quickly as possible on glass plates over ice [22]. The samples were weighed and treated with 1000 µl of an ice-cold 0.2 M tri- chloroacetic acid (TCA) solution containing 0.2 mM sodium pyrosulfite, 0.01% EDTA-2Na, and 0.5 µM iso- proterenol (ISO) and 3-nitro-L-tyrosine (3-NTYR) as an internal standard per 100 mg of wet tissue. The solution was sonicated and then centrifuged at 10,000g for 20 min at 4˚C. The supernatant was filtered through a Mil- lipore HV filter (0.45 µm pore size) and then subjected to both high-performance liquid chromatography (HPLC) with electrochemical detection (ECD) of 5-HT, and HPLC with fluorimetric detection (FD) of TRP as well as ultraviolet (UV) detection of KYN. The standard solution was prepared using the above- mentioned ice-cold 0.2 M TCA solution containing 0.5-µM internal standards (ISO, 3-NTYR), and the solu-
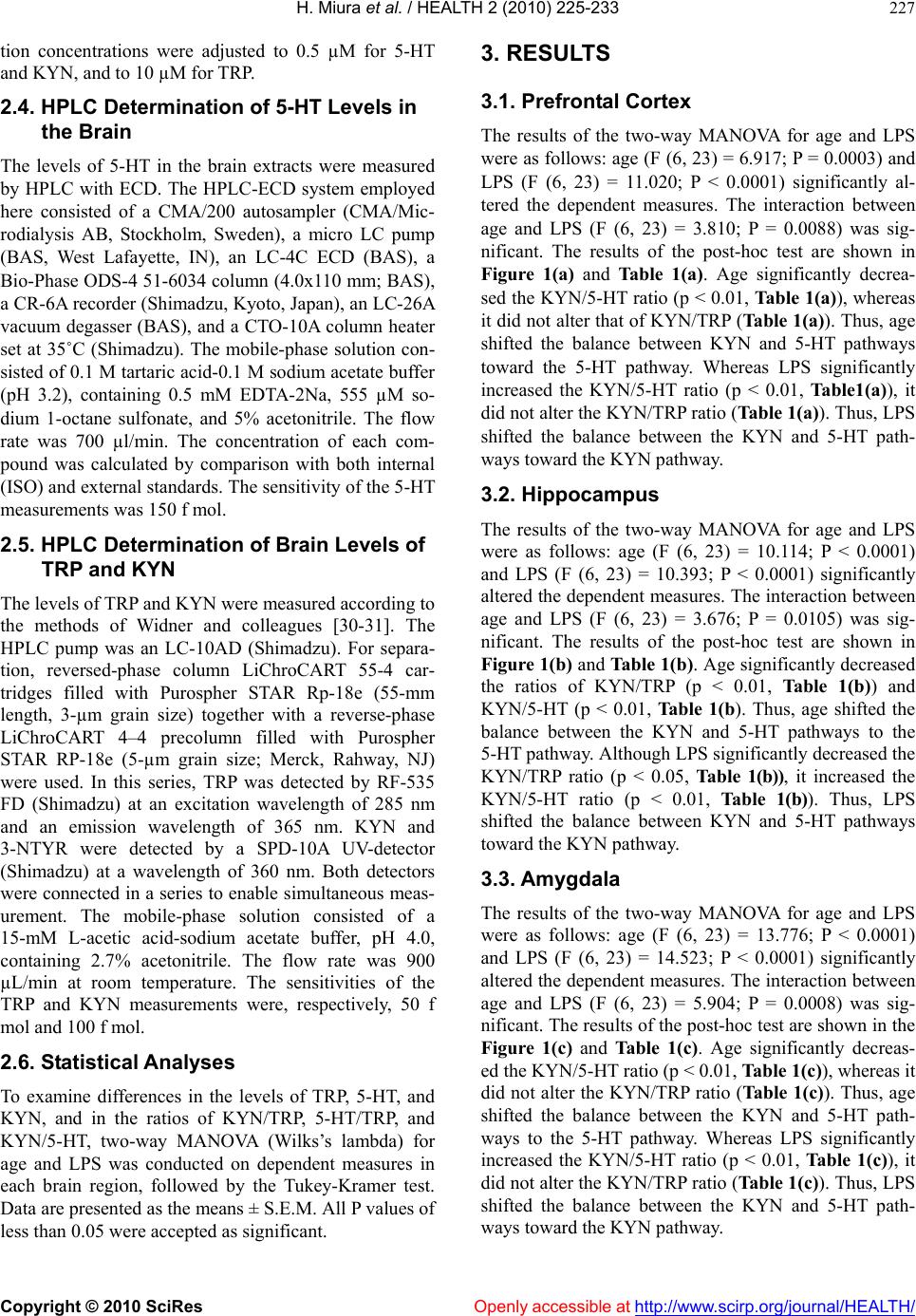 H. Miura et al. / HEALTH 2 (2010) 225-233 Copyright © 2010 SciRes http://www.scirp.org/journal/HEALTH/Openly accessible at 227 tion concentrations were adjusted to 0.5 µM for 5-HT and KYN, and to 10 µM for TRP. 2.4. HPLC Determination of 5-HT Levels in the Brain The levels of 5-HT in the brain extracts were measured by HPLC with ECD. The HPLC-ECD system employed here consisted of a CMA/200 autosampler (CMA/Mic- rodialysis AB, Stockholm, Sweden), a micro LC pump (BAS, West Lafayette, IN), an LC-4C ECD (BAS), a Bio-Phase ODS-4 51-6034 column (4.0x110 mm; BAS), a CR-6A recorder (Shimadzu, Kyoto, Japan), an LC-26A vacuum degasser (BAS), and a CTO-10A column heater set at 35˚C (Shimadzu). The mobile-phase solution con- sisted of 0.1 M tartaric acid-0.1 M sodium acetate buffer (pH 3.2), containing 0.5 mM EDTA-2Na, 555 µM so- dium 1-octane sulfonate, and 5% acetonitrile. The flow rate was 700 µl/min. The concentration of each com- pound was calculated by comparison with both internal (ISO) and external standards. The sensitivity of the 5-HT measurements was 150 f mol. 2.5. HPLC Determination of Brain Levels of TRP and KYN The levels of TRP and KYN were measured according to the methods of Widner and colleagues [30-31]. The HPLC pump was an LC-10AD (Shimadzu). For separa- tion, reversed-phase column LiChroCART 55-4 car- tridges filled with Purospher STAR Rp-18e (55-mm length, 3-µm grain size) together with a reverse-phase LiChroCART 4–4 precolumn filled with Purospher STAR RP-18e (5-µm grain size; Merck, Rahway, NJ) were used. In this series, TRP was detected by RF-535 FD (Shimadzu) at an excitation wavelength of 285 nm and an emission wavelength of 365 nm. KYN and 3-NTYR were detected by a SPD-10A UV-detector (Shimadzu) at a wavelength of 360 nm. Both detectors were connected in a series to enable simultaneous meas- urement. The mobile-phase solution consisted of a 15-mM L-acetic acid-sodium acetate buffer, pH 4.0, containing 2.7% acetonitrile. The flow rate was 900 µL/min at room temperature. The sensitivities of the TRP and KYN measurements were, respectively, 50 f mol and 100 f mol. 2.6. Statistical Analyses To examine differences in the levels of TRP, 5-HT, and KYN, and in the ratios of KYN/TRP, 5-HT/TRP, and KYN/5-HT, two-way MANOVA (Wilks’s lambda) for age and LPS was conducted on dependent measures in each brain region, followed by the Tukey-Kramer test. Data are presented as the means ± S.E.M. All P values of less than 0.05 were accepted as significant. 3. RESULTS 3.1. Prefrontal Cortex The results of the two-way MANOVA for age and LPS were as follows: age (F (6, 23) = 6.917; P = 0.0003) and LPS (F (6, 23) = 11.020; P < 0.0001) significantly al- tered the dependent measures. The interaction between age and LPS (F (6, 23) = 3.810; P = 0.0088) was sig- nificant. The results of the post-hoc test are shown in Figure 1(a) and Table 1(a). Age significantly decrea- sed the KYN/5-HT ratio (p < 0.01, Table 1(a)), whereas it did not alter that of KYN/TRP (Table 1(a)). Thus, age shifted the balance between KYN and 5-HT pathways toward the 5-HT pathway. Whereas LPS significantly increased the KYN/5-HT ratio (p < 0.01, Table1(a)), it did not alter the KYN/TRP ratio (Table 1(a)). Thus, LPS shifted the balance between the KYN and 5-HT path- ways toward the KYN pathway. 3.2. Hippocampus The results of the two-way MANOVA for age and LPS were as follows: age (F (6, 23) = 10.114; P < 0.0001) and LPS (F (6, 23) = 10.393; P < 0.0001) significantly altered the dependent measures. The interaction between age and LPS (F (6, 23) = 3.676; P = 0.0105) was sig- nificant. The results of the post-hoc test are shown in Figure 1(b) and Table 1(b). Age significantly decreased the ratios of KYN/TRP (p < 0.01, Table 1(b)) and KYN/5-HT (p < 0.01, Table 1(b). Thus, age shifted the balance between the KYN and 5-HT pathways to the 5-HT pathway. Although LPS significantly decreased the KYN/TRP ratio (p < 0.05, Table 1(b)), it increased the KYN/5-HT ratio (p < 0.01, Table 1(b)). Thus, LPS shifted the balance between KYN and 5-HT pathways toward the KYN pathway. 3.3. Amygdala The results of the two-way MANOVA for age and LPS were as follows: age (F (6, 23) = 13.776; P < 0.0001) and LPS (F (6, 23) = 14.523; P < 0.0001) significantly altered the dependent measures. The interaction between age and LPS (F (6, 23) = 5.904; P = 0.0008) was sig- nificant. The results of the post-hoc test are shown in the Figure 1(c) and Table 1(c). Age significantly decreas- ed the KYN/5-HT ratio (p < 0.01, Table 1(c)), whereas it did not alter the KYN/TRP ratio (Table 1(c)). Thus, age shifted the balance between the KYN and 5-HT path- ways to the 5-HT pathway. Whereas LPS significantly increased the KYN/5-HT ratio (p < 0.01, Table 1(c)), it did not alter the KYN/TRP ratio (Table 1(c)). Thus, LPS shifted the balance between the KYN and 5-HT path- ays toward the KYN pathway. w
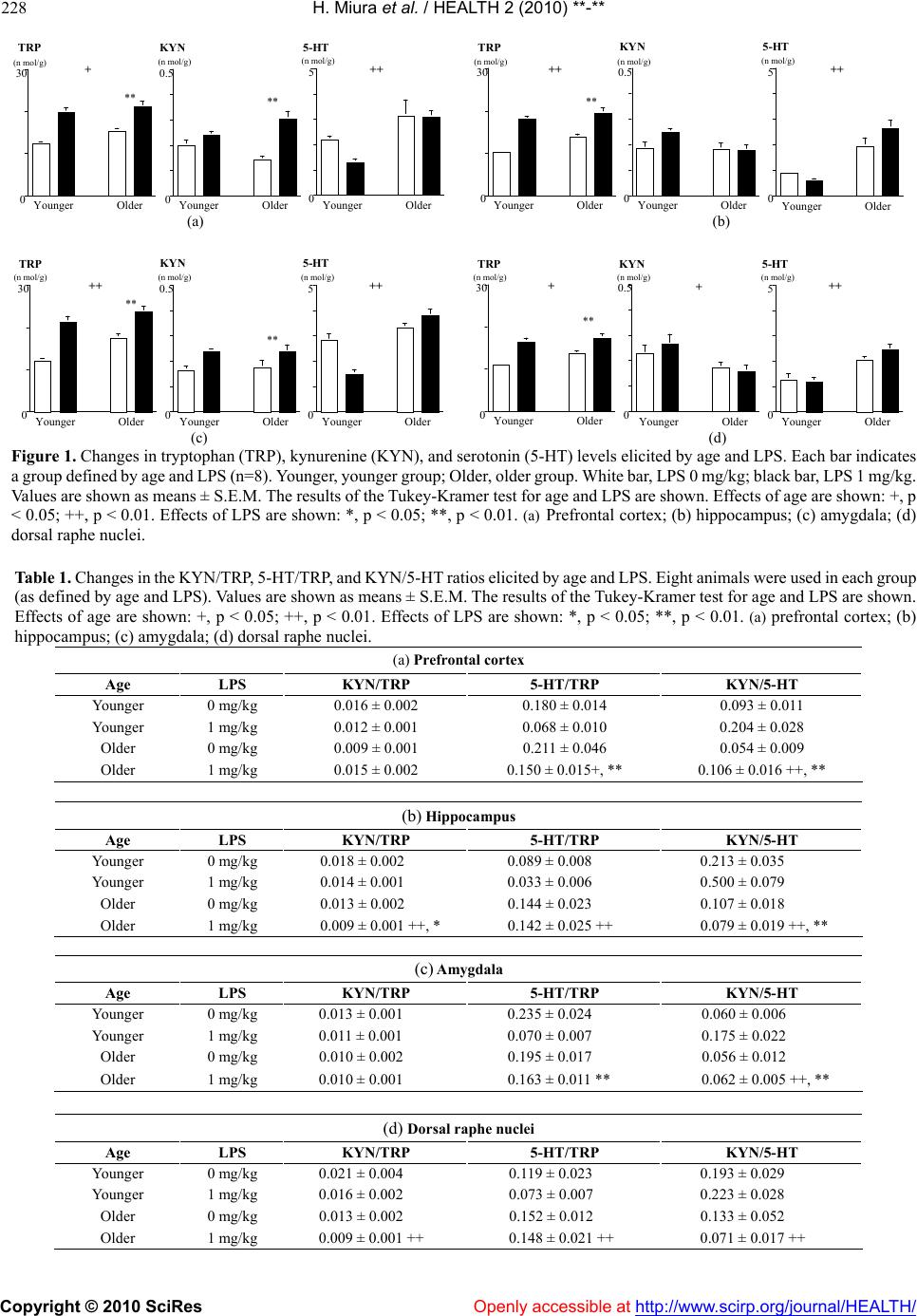 H. Miura et al. / HEALTH 2 (2010) **-** Copyright © 2010 SciRes Openly accessible at http://www.scirp.org/journal/HEALTH/ 228 0 30 Younger Older (n mol/g) 0 0.5 Younger Older (n mol/g) 0 5 Younger Older (n mol/g) TRP KYN 5-HT + ++ ** ** 0 30 Younger Older (n mol/g) 0 0.5 Younger Older (n mol/g) 0 5 Younger Older (n mol/g) TRP KYN5-HT ++ ++ ** (a) (b) 0 30 Younger Older (n mol/g) 0 0.5 Younger Older (n mol/g) 0 5 Younger Older (n mol/g) TRP KYN 5-HT ++ ++ ** ** 0 30 Younger Older (n mol/g) 0 0.5 Younger Older (n mol/g) 0 5 Younger Older (n mol/g) TRPKYN5-HT ++ ++ ** (c) (d) Figure 1. Changes in tryptophan (TRP), kynurenine (KYN), and serotonin (5-HT) levels elicited by age and LPS. Each bar indicates a group defined by age and LPS (n=8). Younger, younger group; Older, older group. White bar, LPS 0 mg/kg; black bar, LPS 1 mg/kg. Values are shown as means ± S.E.M. The results of the Tukey-Kramer test for age and LPS are shown. Effects of age are shown: +, p < 0.05; ++, p < 0.01. Effects of LPS are shown: *, p < 0.05; **, p < 0.01. (a) Prefrontal cortex; (b) hippocampus; (c) amygdala; (d) dorsal raphe nuclei. Table 1. Changes in the KYN/TRP, 5-HT/TRP, and KYN/5-HT ratios elicited by age and LPS. Eight animals were used in each group (as defined by age and LPS). Values are shown as means ± S.E.M. The results of the Tukey-Kramer test for age and LPS are shown. Effects of age are shown: +, p < 0.05; ++, p < 0.01. Effects of LPS are shown: *, p < 0.05; **, p < 0.01. (a) prefrontal cortex; (b) hippocampus; (c) amygdala; (d) dorsal raphe nuclei. (a) Prefrontal cortex Age LPS KYN/TRP 5-HT/TRP KYN/5-HT Younger 0 mg/kg 0.016 ± 0.002 0.180 ± 0.014 0.093 ± 0.011 Younger 1 mg/kg 0.012 ± 0.001 0.068 ± 0.010 0.204 ± 0.028 Older 0 mg/kg 0.009 ± 0.001 0.211 ± 0.046 0.054 ± 0.009 Older 1 mg/kg 0.015 ± 0.002 0.150 ± 0.015+, ** 0.106 ± 0.016 ++, ** (b) Hippocampus Age LPS KYN/TRP 5-HT/TRP KYN/5-HT Younger 0 mg/kg 0.018 ± 0.002 0.089 ± 0.008 0.213 ± 0.035 Younger 1 mg/kg 0.014 ± 0.001 0.033 ± 0.006 0.500 ± 0.079 Older 0 mg/kg 0.013 ± 0.002 0.144 ± 0.023 0.107 ± 0.018 Older 1 mg/kg 0.009 ± 0.001 ++, * 0.142 ± 0.025 ++ 0.079 ± 0.019 ++, ** (c) Amygdala Age LPS KYN/TRP 5-HT/TRP KYN/5-HT Younger 0 mg/kg 0.013 ± 0.001 0.235 ± 0.024 0.060 ± 0.006 Younger 1 mg/kg 0.011 ± 0.001 0.070 ± 0.007 0.175 ± 0.022 Older 0 mg/kg 0.010 ± 0.002 0.195 ± 0.017 0.056 ± 0.012 Older 1 mg/kg 0.010 ± 0.001 0.163 ± 0.011 ** 0.062 ± 0.005 ++, ** (d) Dorsal raphe nuclei Age LPS KYN/TRP 5-HT/TRP KYN/5-HT Younger 0 mg/kg 0.021 ± 0.004 0.119 ± 0.023 0.193 ± 0.029 Younger 1 mg/kg 0.016 ± 0.002 0.073 ± 0.007 0.223 ± 0.028 Older 0 mg/kg 0.013 ± 0.002 0.152 ± 0.012 0.133 ± 0.052 Older 1 mg/kg 0.009 ± 0.001 ++ 0.148 ± 0.021 ++ 0.071 ± 0.017 ++
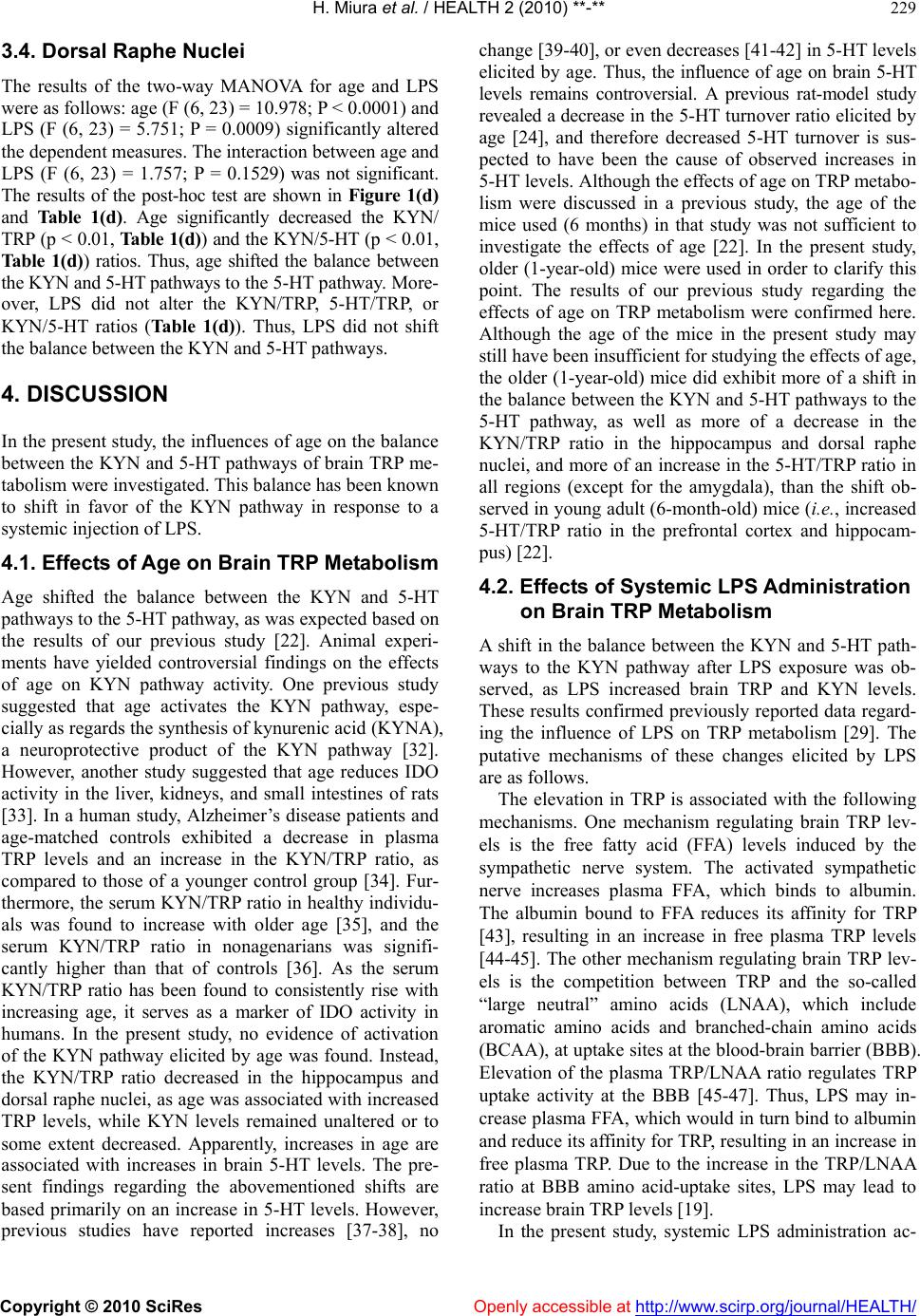 H. Miura et al. / HEALTH 2 (2010) **-** Copyright © 2010 SciRes Openly accessible at http://www.scirp.org/journal/HEALTH/ 229 3.4. Dorsal Raphe Nuclei The results of the two-way MANOVA for age and LPS were as follows: age (F (6, 23) = 10.978; P < 0.0001) and LPS (F (6, 23) = 5.751; P = 0.0009) significantly altered the dependent measures. The interaction between age and LPS (F (6, 23) = 1.757; P = 0.1529) was not significant. The results of the post-hoc test are shown in Figure 1(d) and Table 1(d). Age significantly decreased the KYN/ TRP (p < 0.01, Table 1(d)) and the KYN/5-HT (p < 0.01, Table 1(d)) ratios. Thus, age shifted the balance between the KYN and 5-HT pathways to the 5-HT pathway. More- over, LPS did not alter the KYN/TRP, 5-HT/TRP, or KYN/5-HT ratios (Table 1(d)). Thus, LPS did not shift the balance between the KYN and 5-HT pathways. 4. DISCUSSION In the present study, the influences of age on the balance between the KYN and 5-HT pathways of brain TRP me- tabolism were investigated. This balance has been known to shift in favor of the KYN pathway in response to a systemic injection of LPS. 4.1. Effects of Age on Brain TRP Metabolism Age shifted the balance between the KYN and 5-HT pathways to the 5-HT pathway, as was expected based on the results of our previous study [22]. Animal experi- ments have yielded controversial findings on the effects of age on KYN pathway activity. One previous study suggested that age activates the KYN pathway, espe- cially as regards the synthesis of kynurenic acid (KYNA), a neuroprotective product of the KYN pathway [32]. However, another study suggested that age reduces IDO activity in the liver, kidneys, and small intestines of rats [33]. In a human study, Alzheimer’s disease patients and age-matched controls exhibited a decrease in plasma TRP levels and an increase in the KYN/TRP ratio, as compared to those of a younger control group [34]. Fur- thermore, the serum KYN/TRP ratio in healthy individu- als was found to increase with older age [35], and the serum KYN/TRP ratio in nonagenarians was signifi- cantly higher than that of controls [36]. As the serum KYN/TRP ratio has been found to consistently rise with increasing age, it serves as a marker of IDO activity in humans. In the present study, no evidence of activation of the KYN pathway elicited by age was found. Instead, the KYN/TRP ratio decreased in the hippocampus and dorsal raphe nuclei, as age was associated with increased TRP levels, while KYN levels remained unaltered or to some extent decreased. Apparently, increases in age are associated with increases in brain 5-HT levels. The pre- sent findings regarding the abovementioned shifts are based primarily on an increase in 5-HT levels. However, previous studies have reported increases [37-38], no change [39-40], or even decreases [41-42] in 5-HT levels elicited by age. Thus, the influence of age on brain 5-HT levels remains controversial. A previous rat-model study revealed a decrease in the 5-HT turnover ratio elicited by age [24], and therefore decreased 5-HT turnover is sus- pected to have been the cause of observed increases in 5-HT levels. Although the effects of age on TRP metabo- lism were discussed in a previous study, the age of the mice used (6 months) in that study was not sufficient to investigate the effects of age [22]. In the present study, older (1-year-old) mice were used in order to clarify this point. The results of our previous study regarding the effects of age on TRP metabolism were confirmed here. Although the age of the mice in the present study may still have been insufficient for studying the effects of age, the older (1-year-old) mice did exhibit more of a shift in the balance between the KYN and 5-HT pathways to the 5-HT pathway, as well as more of a decrease in the KYN/TRP ratio in the hippocampus and dorsal raphe nuclei, and more of an increase in the 5-HT/TRP ratio in all regions (except for the amygdala), than the shift ob- served in young adult (6-month-old) mice (i.e., increased 5-HT/TRP ratio in the prefrontal cortex and hippocam- pus) [22]. 4.2. Effects of Systemic LPS Administration on Brain TRP Metabolism A shift in the balance between the KYN and 5-HT path- ways to the KYN pathway after LPS exposure was ob- served, as LPS increased brain TRP and KYN levels. These results confirmed previously reported data regard- ing the influence of LPS on TRP metabolism [29]. The putative mechanisms of these changes elicited by LPS are as follows. The elevation in TRP is associated with the following mechanisms. One mechanism regulating brain TRP lev- els is the free fatty acid (FFA) levels induced by the sympathetic nerve system. The activated sympathetic nerve increases plasma FFA, which binds to albumin. The albumin bound to FFA reduces its affinity for TRP [43], resulting in an increase in free plasma TRP levels [44-45]. The other mechanism regulating brain TRP lev- els is the competition between TRP and the so-called “large neutral” amino acids (LNAA), which include aromatic amino acids and branched-chain amino acids (BCAA), at uptake sites at the blood-brain barrier (BBB). Elevation of the plasma TRP/LNAA ratio regulates TRP uptake activity at the BBB [45-47]. Thus, LPS may in- crease plasma FFA, which would in turn bind to albumin and reduce its affinity for TRP, resulting in an increase in free plasma TRP. Due to the increase in the TRP/LNAA ratio at BBB amino acid-uptake sites, LPS may lead to increase brain TRP levels [19]. In the present study, systemic LPS administration ac-
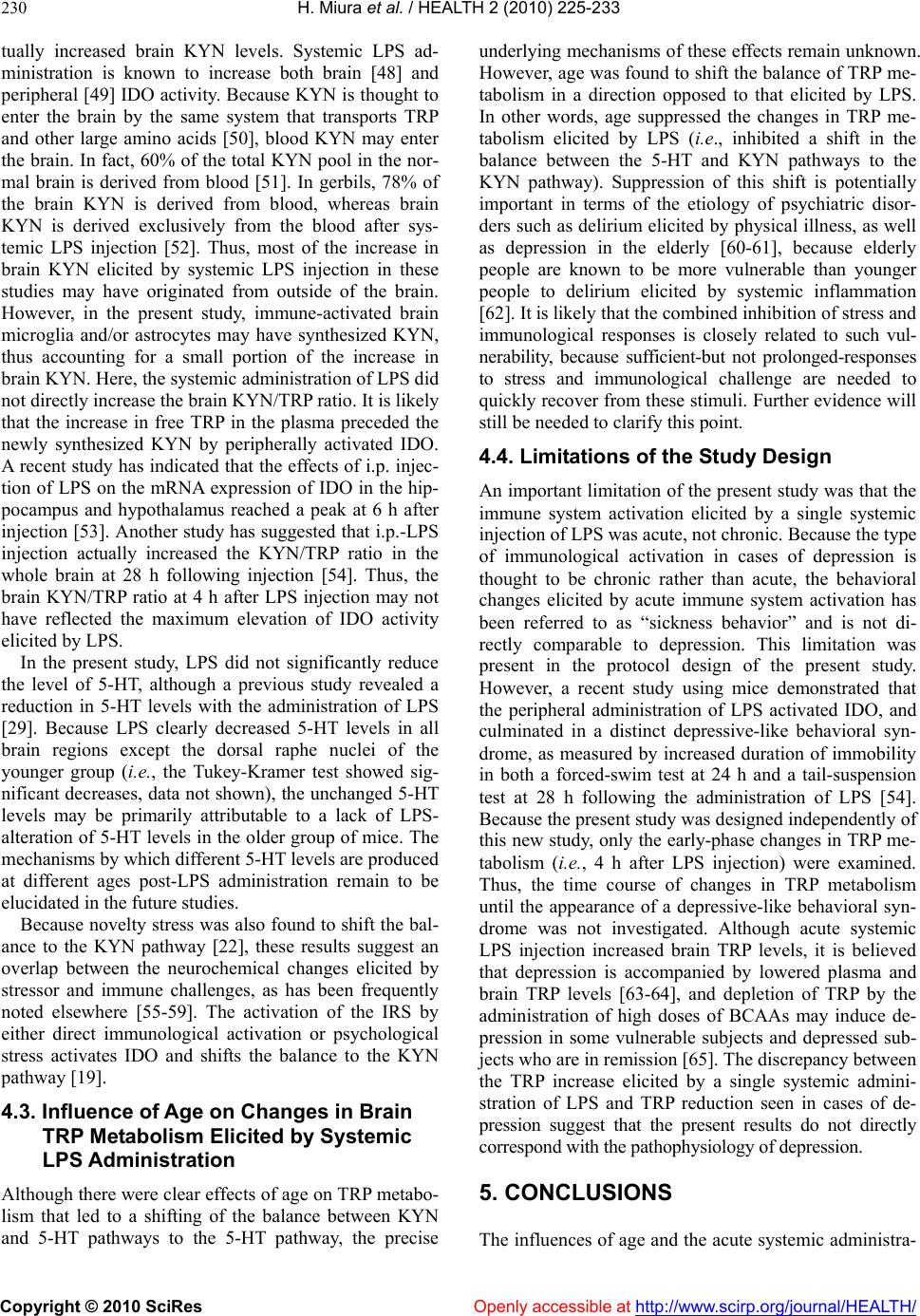 H. Miura et al. / HEALTH 2 (2010) 225-233 Copyright © 2010 SciRes Openly accessible at http://www.scirp.org/journal/HEALTH/ 230 tually increased brain KYN levels. Systemic LPS ad- ministration is known to increase both brain [48] and peripheral [49] IDO activity. Because KYN is thought to enter the brain by the same system that transports TRP and other large amino acids [50], blood KYN may enter the brain. In fact, 60% of the total KYN pool in the nor- mal brain is derived from blood [51]. In gerbils, 78% of the brain KYN is derived from blood, whereas brain KYN is derived exclusively from the blood after sys- temic LPS injection [52]. Thus, most of the increase in brain KYN elicited by systemic LPS injection in these studies may have originated from outside of the brain. However, in the present study, immune-activated brain microglia and/or astrocytes may have synthesized KYN, thus accounting for a small portion of the increase in brain KYN. Here, the systemic administration of LPS did not directly increase the brain KYN/TRP ratio. It is likely that the increase in free TRP in the plasma preceded the newly synthesized KYN by peripherally activated IDO. A recent study has indicated that the effects of i.p. injec- tion of LPS on the mRNA expression of IDO in the hip- pocampus and hypothalamus reached a peak at 6 h after injection [53]. Another study has suggested that i.p.-LPS injection actually increased the KYN/TRP ratio in the whole brain at 28 h following injection [54]. Thus, the brain KYN/TRP ratio at 4 h after LPS injection may not have reflected the maximum elevation of IDO activity elicited by LPS. In the present study, LPS did not significantly reduce the level of 5-HT, although a previous study revealed a reduction in 5-HT levels with the administration of LPS [29]. Because LPS clearly decreased 5-HT levels in all brain regions except the dorsal raphe nuclei of the younger group (i.e., the Tukey-Kramer test showed sig- nificant decreases, data not shown), the unchanged 5-HT levels may be primarily attributable to a lack of LPS- alteration of 5-HT levels in the older group of mice. The mechanisms by which different 5-HT levels are produced at different ages post-LPS administration remain to be elucidated in the future studies. Because novelty stress was also found to shift the bal- ance to the KYN pathway [22], these results suggest an overlap between the neurochemical changes elicited by stressor and immune challenges, as has been frequently noted elsewhere [55-59]. The activation of the IRS by either direct immunological activation or psychological stress activates IDO and shifts the balance to the KYN pathway [19]. 4.3. Influence of Age on Changes in Brain TRP Metabolism Elicited by Systemic LPS Administration Although there were clear effects of age on TRP metabo- lism that led to a shifting of the balance between KYN and 5-HT pathways to the 5-HT pathway, the precise underlying mechanisms of these effects remain unknown. However, age was found to shift the balance of TRP me- tabolism in a direction opposed to that elicited by LPS. In other words, age suppressed the changes in TRP me- tabolism elicited by LPS (i.e., inhibited a shift in the balance between the 5-HT and KYN pathways to the KYN pathway). Suppression of this shift is potentially important in terms of the etiology of psychiatric disor- ders such as delirium elicited by physical illness, as well as depression in the elderly [60-61], because elderly people are known to be more vulnerable than younger people to delirium elicited by systemic inflammation [62]. It is likely that the combined inhibition of stress and immunological responses is closely related to such vul- nerability, because sufficient-but not prolonged-responses to stress and immunological challenge are needed to quickly recover from these stimuli. Further evidence will still be needed to clarify this point. 4.4. Limitations of the Study Design An important limitation of the present study was that the immune system activation elicited by a single systemic injection of LPS was acute, not chronic. Because the type of immunological activation in cases of depression is thought to be chronic rather than acute, the behavioral changes elicited by acute immune system activation has been referred to as “sickness behavior” and is not di- rectly comparable to depression. This limitation was present in the protocol design of the present study. However, a recent study using mice demonstrated that the peripheral administration of LPS activated IDO, and culminated in a distinct depressive-like behavioral syn- drome, as measured by increased duration of immobility in both a forced-swim test at 24 h and a tail-suspension test at 28 h following the administration of LPS [54]. Because the present study was designed independently of this new study, only the early-phase changes in TRP me- tabolism (i.e., 4 h after LPS injection) were examined. Thus, the time course of changes in TRP metabolism until the appearance of a depressive-like behavioral syn- drome was not investigated. Although acute systemic LPS injection increased brain TRP levels, it is believed that depression is accompanied by lowered plasma and brain TRP levels [63-64], and depletion of TRP by the administration of high doses of BCAAs may induce de- pression in some vulnerable subjects and depressed sub- jects who are in remission [65]. The discrepancy between the TRP increase elicited by a single systemic admini- stration of LPS and TRP reduction seen in cases of de- pression suggest that the present results do not directly correspond with the pathophysiology of depression. 5. CONCLUSIONS The influences of age and the acute systemic administra-
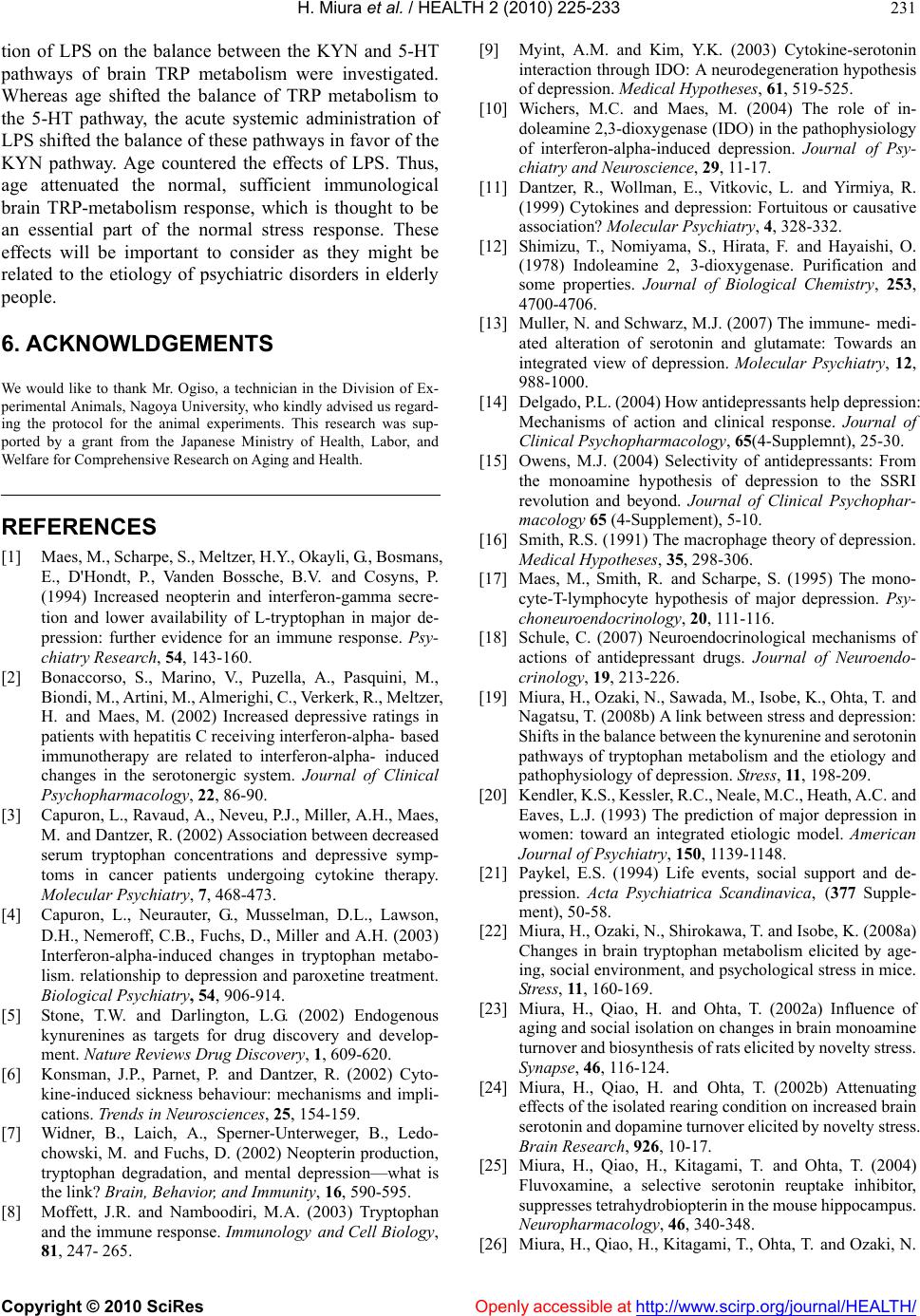 H. Miura et al. / HEALTH 2 (2010) 225-233 Copyright © 2010 SciRes Openly accessible at http://www.scirp.org/journal/HEALTH/ 231 tion of LPS on the balance between the KYN and 5-HT pathways of brain TRP metabolism were investigated. Whereas age shifted the balance of TRP metabolism to the 5-HT pathway, the acute systemic administration of LPS shifted the balance of these pathways in favor of the KYN pathway. Age countered the effects of LPS. Thus, age attenuated the normal, sufficient immunological brain TRP-metabolism response, which is thought to be an essential part of the normal stress response. These effects will be important to consider as they might be related to the etiology of psychiatric disorders in elderly people. 6. ACKNOWLDGEMENTS We would like to thank Mr. Ogiso, a technician in the Division of Ex- perimental Animals, Nagoya University, who kindly advised us regard- ing the protocol for the animal experiments. This research was sup- ported by a grant from the Japanese Ministry of Health, Labor, and Welfare for Comprehensive Research on Aging and Health. REFERENCES [1] Maes, M., Scharpe, S., Meltzer, H.Y., Okayli, G., Bosmans, E., D'Hondt, P., Vanden Bossche, B.V. and Cosyns, P. (1994) Increased neopterin and interferon-gamma secre- tion and lower availability of L-tryptophan in major de- pression: further evidence for an immune response. Psy- chiatry Research, 54, 143-160. [2] Bonaccorso, S., Marino, V., Puzella, A., Pasquini, M., Biondi, M., Artini, M., Almerighi, C., Verkerk, R., Meltzer, H. and Maes, M. (2002) Increased depressive ratings in patients with hepatitis C receiving interferon-alpha- based immunotherapy are related to interferon-alpha- induced changes in the serotonergic system. Journal of Clinical Psychopharmacology, 22, 86-90. [3] Capuron, L., Ravaud, A., Neveu, P.J., Miller, A.H., Maes, M. and Dantzer, R. (2002) Association between decreased serum tryptophan concentrations and depressive symp- toms in cancer patients undergoing cytokine therapy. Molecular Psychiatry, 7, 468-473. [4] Capuron, L., Neurauter, G., Musselman, D.L., Lawson, D.H., Nemeroff, C.B., Fuchs, D., Miller and A.H. (2003) Interferon-alpha-induced changes in tryptophan metabo- lism. relationship to depression and paroxetine treatment. Biological Psychiatry, 54, 906-914. [5] Stone, T.W. and Darlington, L.G. (2002) Endogenous kynurenines as targets for drug discovery and develop- ment. Nature Reviews Drug Discovery, 1, 609-620. [6] Konsman, J.P., Parnet, P. and Dantzer, R. (2002) Cyto- kine-induced sickness behaviour: mechanisms and impli- cations. Trends in Neurosciences, 25, 154-159. [7] Widner, B., Laich, A., Sperner-Unterweger, B., Ledo- chowski, M. and Fuchs, D. (2002) Neopterin production, tryptophan degradation, and mental depression—what is the link? Brain, Behavior, and Immunity, 16, 590-595. [8] Moffett, J.R. and Namboodiri, M.A. (2003) Tryptophan and the immune response. Immunology and Cell Biology, 81, 247- 265. [9] Myint, A.M. and Kim, Y.K. (2003) Cytokine-serotonin interaction through IDO: A neurodegeneration hypothesis of depression. Medical Hypotheses, 61, 519-525. [10] Wichers, M.C. and Maes, M. (2004) The role of in- doleamine 2,3-dioxygenase (IDO) in the pathophysiology of interferon-alpha-induced depression. Journal of Psy- chiatry and Neuroscience, 29, 11-17. [11] Dantzer, R., Wollman, E., Vitkovic, L. and Yirmiya, R. (1999) Cytokines and depression: Fortuitous or causative association? Molecular Psychiatry, 4, 328-332. [12] Shimizu, T., Nomiyama, S., Hirata, F. and Hayaishi, O. (1978) Indoleamine 2, 3-dioxygenase. Purification and some properties. Journal of Biological Chemistry, 253, 4700-4706. [13] Muller, N. and Schwarz, M.J. (2007) The immune- medi- ated alteration of serotonin and glutamate: Towards an integrated view of depression. Molecular Psychiatry, 12, 988-1000. [14] Delgado, P.L. (2004) How antidepressants help depression: Mechanisms of action and clinical response. Journal of Clinical Psychopharmacology, 65(4-Supplemnt), 25-30. [15] Owens, M.J. (2004) Selectivity of antidepressants: From the monoamine hypothesis of depression to the SSRI revolution and beyond. Journal of Clinical Psychophar- macology 65 (4-Supplement), 5-10. [16] Smith, R.S. (1991) The macrophage theory of depression. Medical Hypotheses, 35, 298-306. [17] Maes, M., Smith, R. and Scharpe, S. (1995) The mono- cyte-T-lymphocyte hypothesis of major depression. Psy- choneuroendocrinology, 20, 111-116. [18] Schule, C. (2007) Neuroendocrinological mechanisms of actions of antidepressant drugs. Journal of Neuroendo- crinology, 19, 213-226. [19] Miura, H., Ozaki, N., Sawada, M., Isobe, K., Ohta, T. and Nagatsu, T. (2008b) A link between stress and depression: Shifts in the balance between the kynurenine and serotonin pathways of tryptophan metabolism and the etiology and pathophysiology of depression. Stress, 11, 198-209. [20] Kendler, K.S., Kessler, R.C., Neale, M.C., Heath, A.C. and Eaves, L.J. (1993) The prediction of major depression in women: toward an integrated etiologic model. American Journal of Psychiatry, 150, 1139-1148. [21] Paykel, E.S. (1994) Life events, social support and de- pression. Acta Psychiatrica Scandinavica, (377 Supple- ment), 50-58. [22] Miura, H., Ozaki, N., Shirokawa, T. and Isobe, K. (2008a) Changes in brain tryptophan metabolism elicited by age- ing, social environment, and psychological stress in mice. St ress, 11, 160-169. [23] Miura, H., Qiao, H. and Ohta, T. (2002a) Influence of aging and social isolation on changes in brain monoamine turnover and biosynthesis of rats elicited by novelty stress. Synapse, 46, 116-124. [24] Miura, H., Qiao, H. and Ohta, T. (2002b) Attenuating effects of the isolated rearing condition on increased brain serotonin and dopamine turnover elicited by novelty stress. Brain Research, 926, 10-17. [25] Miura, H., Qiao, H., Kitagami, T. and Ohta, T. (2004) Fluvoxamine, a selective serotonin reuptake inhibitor, suppresses tetrahydrobiopterin in the mouse hippocampus. Neuropharmacology, 46, 340-348. [26] Miura, H., Qiao, H., Kitagami, T., Ohta, T. and Ozaki, N.
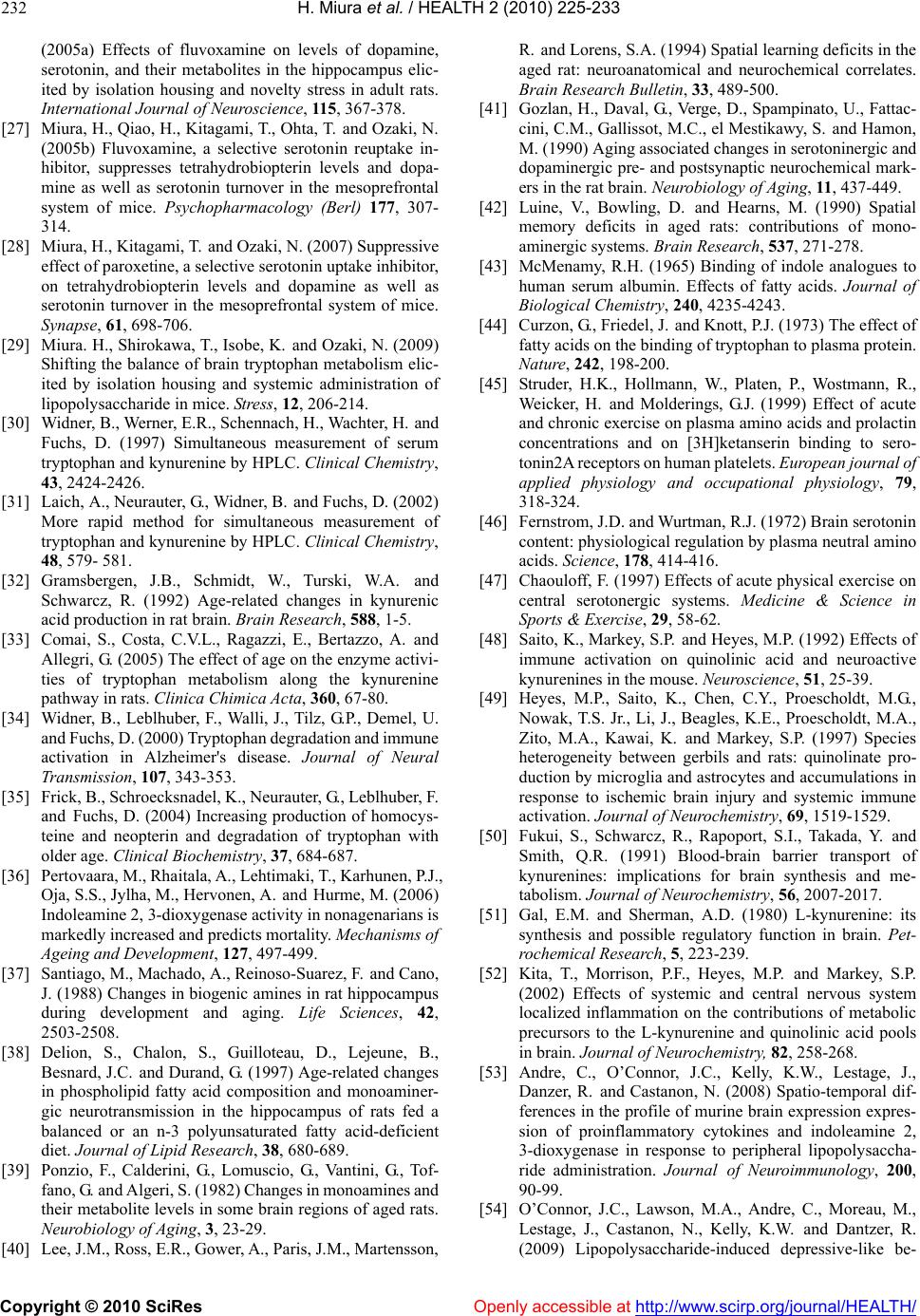 H. Miura et al. / HEALTH 2 (2010) 225-233 Copyright © 2010 SciRes Openly accessible at http://www.scirp.org/journal/HEALTH/ 232 (2005a) Effects of fluvoxamine on levels of dopamine, serotonin, and their metabolites in the hippocampus elic- ited by isolation housing and novelty stress in adult rats. International Journal of Neuroscience, 115, 367-378. [27] Miura, H., Qiao, H., Kitagami, T., Ohta, T. and Ozaki, N. (2005b) Fluvoxamine, a selective serotonin reuptake in- hibitor, suppresses tetrahydrobiopterin levels and dopa- mine as well as serotonin turnover in the mesoprefrontal system of mice. Psychopharmacology (Berl) 177, 307- 314. [28] Miura, H., Kitagami, T. and Ozaki, N. (2007) Suppressive effect of paroxetine, a selective serotonin uptake inhibitor, on tetrahydrobiopterin levels and dopamine as well as serotonin turnover in the mesoprefrontal system of mice. Synapse, 61, 698-706. [29] Miura. H., Shirokawa, T., Isobe, K. and Ozaki, N. (2009) Shifting the balance of brain tryptophan metabolism elic- ited by isolation housing and systemic administration of lipopolysaccharide in mice. Stress, 12, 206-214. [30] Widner, B., Werner, E.R., Schennach, H., Wachter, H. and Fuchs, D. (1997) Simultaneous measurement of serum tryptophan and kynurenine by HPLC. Clinical Chemistry, 43, 2424-2426. [31] Laich, A., Neurauter, G., Widner, B. and Fuchs, D. (2002) More rapid method for simultaneous measurement of tryptophan and kynurenine by HPLC. Clinical Chemistry, 48, 579- 581. [32] Gramsbergen, J.B., Schmidt, W., Turski, W.A. and Schwarcz, R. (1992) Age-related changes in kynurenic acid production in rat brain. Brain Research, 588, 1-5. [33] Comai, S., Costa, C.V.L., Ragazzi, E., Bertazzo, A. and Allegri, G. (2005) The effect of age on the enzyme activi- ties of tryptophan metabolism along the kynurenine pathway in rats. Clinica Chimica Acta, 360, 67-80. [34] Widner, B., Leblhuber, F., Walli, J., Tilz, G.P., Demel, U. and Fuchs, D. (2000) Tryptophan degradation and immune activation in Alzheimer's disease. Journal of Neural Transmission, 107 , 343-353. [35] Frick, B., Schroecksnadel, K., Neurauter, G., Leblhuber, F. and Fuchs, D. (2004) Increasing production of homocys- teine and neopterin and degradation of tryptophan with older age. Clinical Biochemistry, 37, 684-687. [36] Pertovaara, M., Rhaitala, A., Lehtimaki, T., Karhunen, P.J., Oja, S.S., Jylha, M., Hervonen, A. and Hurme, M. (2006) Indoleamine 2, 3-dioxygenase activity in nonagenarians is markedly increased and predicts mortality. Mechanisms of Ageing and Development, 127, 497-499. [37] Santiago, M., Machado, A., Reinoso-Suarez, F. and Cano, J. (1988) Changes in biogenic amines in rat hippocampus during development and aging. Life Sciences, 42, 2503-2508. [38] Delion, S., Chalon, S., Guilloteau, D., Lejeune, B., Besnard, J.C. and Durand, G. (1997) Age-related changes in phospholipid fatty acid composition and monoaminer- gic neurotransmission in the hippocampus of rats fed a balanced or an n-3 polyunsaturated fatty acid-deficient diet. Journal of Lipid Research, 38, 680-689. [39] Ponzio, F., Calderini, G., Lomuscio, G., Vantini, G., Tof- fano, G. and Algeri, S. (1982) Changes in monoamines and their metabolite levels in some brain regions of aged rats. Neurobiology of Aging, 3, 23-29. [40] Lee, J.M., Ross, E.R., Gower, A., Paris, J.M., Martensson, R. and Lorens, S.A. (1994) Spatial learning deficits in the aged rat: neuroanatomical and neurochemical correlates. Brain Research Bulletin, 33, 489-500. [41] Gozlan, H., Daval, G., Verge, D., Spampinato, U., Fattac- cini, C.M., Gallissot, M.C., el Mestikawy, S. and Hamon, M. (1990) Aging associated changes in serotoninergic and dopaminergic pre- and postsynaptic neurochemical mark- ers in the rat brain. Neurobiology of Aging, 11, 437-449. [42] Luine, V., Bowling, D. and Hearns, M. (1990) Spatial memory deficits in aged rats: contributions of mono- aminergic systems. Brain Research, 537, 271-278. [43] McMenamy, R.H. (1965) Binding of indole analogues to human serum albumin. Effects of fatty acids. Journal of Biological Chemistry, 240, 4235-4243. [44] Curzon, G., Friedel, J. and Knott, P.J. (1973) The effect of fatty acids on the binding of tryptophan to plasma protein. Nature, 242, 198-200. [45] Struder, H.K., Hollmann, W., Platen, P., Wostmann, R., Weicker, H. and Molderings, G.J. (1999) Effect of acute and chronic exercise on plasma amino acids and prolactin concentrations and on [3H]ketanserin binding to sero- tonin2A receptors on human platelets. European journal of applied physiology and occupational physiology, 79, 318-324. [46] Fernstrom, J.D. and Wurtman, R.J. (1972) Brain serotonin content: physiological regulation by plasma neutral amino acids. Science, 178, 414-416. [47] Chaouloff, F. (1997) Effects of acute physical exercise on central serotonergic systems. Medicine & Science in Sports & Exercise, 29, 58-62. [48] Saito, K., Markey, S.P. and Heyes, M.P. (1992) Effects of immune activation on quinolinic acid and neuroactive kynurenines in the mouse. Neuroscience, 51, 25-39. [49] Heyes, M.P., Saito, K., Chen, C.Y., Proescholdt, M.G., Nowak, T.S. Jr., Li, J., Beagles, K.E., Proescholdt, M.A., Zito, M.A., Kawai, K. and Markey, S.P. (1997) Species heterogeneity between gerbils and rats: quinolinate pro- duction by microglia and astrocytes and accumulations in response to ischemic brain injury and systemic immune activation. Journal of Neurochemistry, 69, 1519-1529. [50] Fukui, S., Schwarcz, R., Rapoport, S.I., Takada, Y. and Smith, Q.R. (1991) Blood-brain barrier transport of kynurenines: implications for brain synthesis and me- tabolism. Journal of Neurochemistry, 56, 2007-2017. [51] Gal, E.M. and Sherman, A.D. (1980) L-kynurenine: its synthesis and possible regulatory function in brain. Pet- rochemical Research, 5, 223-239. [52] Kita, T., Morrison, P.F., Heyes, M.P. and Markey, S.P. (2002) Effects of systemic and central nervous system localized inflammation on the contributions of metabolic precursors to the L-kynurenine and quinolinic acid pools in brain. Journal of Neurochemistry, 82, 258-268. [53] Andre, C., O’Connor, J.C., Kelly, K.W., Lestage, J., Danzer, R. and Castanon, N. (2008) Spatio-temporal dif- ferences in the profile of murine brain expression expres- sion of proinflammatory cytokines and indoleamine 2, 3-dioxygenase in response to peripheral lipopolysaccha- ride administration. Journal of Neuroimmunology, 200, 90-99. [54] O’Connor, J.C., Lawson, M.A., Andre, C., Moreau, M., Lestage, J., Castanon, N., Kelly, K.W. and Dantzer, R. (2009) Lipopolysaccharide-induced depressive-like be-
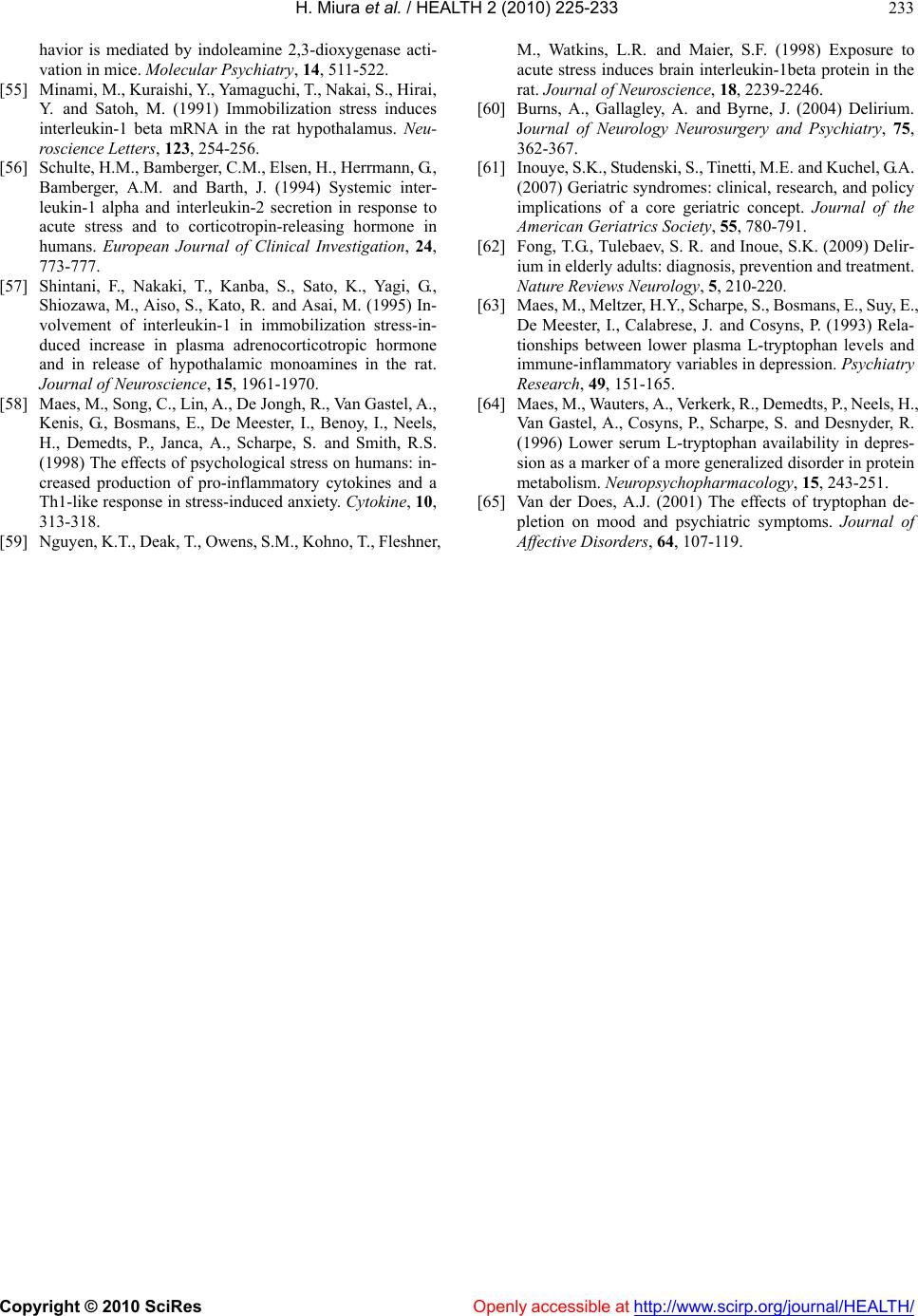 H. Miura et al. / HEALTH 2 (2010) 225-233 Copyright © 2010 SciRes http://www.scirp.org/journal/HEALTH/Openly accessible at 233 havior is mediated by indoleamine 2,3-dioxygenase acti- vation in mice. Molecular Psychiatry, 14, 511-522. [55] Minami, M., Kuraishi, Y., Yamaguchi, T., Nakai, S., Hirai, Y. and Satoh, M. (1991) Immobilization stress induces interleukin-1 beta mRNA in the rat hypothalamus. Neu- roscience Letters, 123, 254-256. [56] Schulte, H.M., Bamberger, C.M., Elsen, H., Herrmann, G., Bamberger, A.M. and Barth, J. (1994) Systemic inter- leukin-1 alpha and interleukin-2 secretion in response to acute stress and to corticotropin-releasing hormone in humans. European Journal of Clinical Investigation, 24, 773-777. [57] Shintani, F., Nakaki, T., Kanba, S., Sato, K., Yagi, G., Shiozawa, M., Aiso, S., Kato, R. and Asai, M. (1995) In- volvement of interleukin-1 in immobilization stress-in- duced increase in plasma adrenocorticotropic hormone and in release of hypothalamic monoamines in the rat. Journal of Neuroscience, 15, 1961-1970. [58] Maes, M., Song, C., Lin, A., De Jongh, R., Van Gastel, A., Kenis, G., Bosmans, E., De Meester, I., Benoy, I., Neels, H., Demedts, P., Janca, A., Scharpe, S. and Smith, R.S. (1998) The effects of psychological stress on humans: in- creased production of pro-inflammatory cytokines and a Th1-like response in stress-induced anxiety. Cyt okin e, 10, 313-318. [59] Nguyen, K.T., Deak, T., Owens, S.M., Kohno, T., Fleshner, M., Watkins, L.R. and Maier, S.F. (1998) Exposure to acute stress induces brain interleukin-1beta protein in the rat. Journal of Neuroscience, 18, 2239-2246. [60] Burns, A., Gallagley, A. and Byrne, J. (2004) Delirium. Journal of Neurology Neurosurgery and Psychiatry, 75, 362-367. [61] Inouye, S.K., Studenski, S., Tinetti, M.E. and Kuchel, G.A. (2007) Geriatric syndromes: clinical, research, and policy implications of a core geriatric concept. Journal of the American Geriatrics Society, 55, 780-791. [62] Fong, T.G., Tulebaev, S. R. and Inoue, S.K. (2009) Delir- ium in elderly adults: diagnosis, prevention and treatment. Nature Reviews Neurology, 5, 210-220. [63] Maes, M., Meltzer, H.Y., Scharpe, S., Bosmans, E., Suy, E., De Meester, I., Calabrese, J. and Cosyns, P. (1993) Rela- tionships between lower plasma L-tryptophan levels and immune-inflammatory variables in depression. Psychiatry Research, 49, 151-165. [64] Maes, M., Wauters, A., Verkerk, R., Demedts, P., Neels, H., Van Gastel, A., Cosyns, P., Scharpe, S. and Desnyder, R. (1996) Lower serum L-tryptophan availability in depres- sion as a marker of a more generalized disorder in protein metabolism. Neuropsychopharmacology, 15, 243-251. [65] Van der Does, A.J. (2001) The effects of tryptophan de- pletion on mood and psychiatric symptoms. Journal of Affective Disorders, 64, 107-119.
|