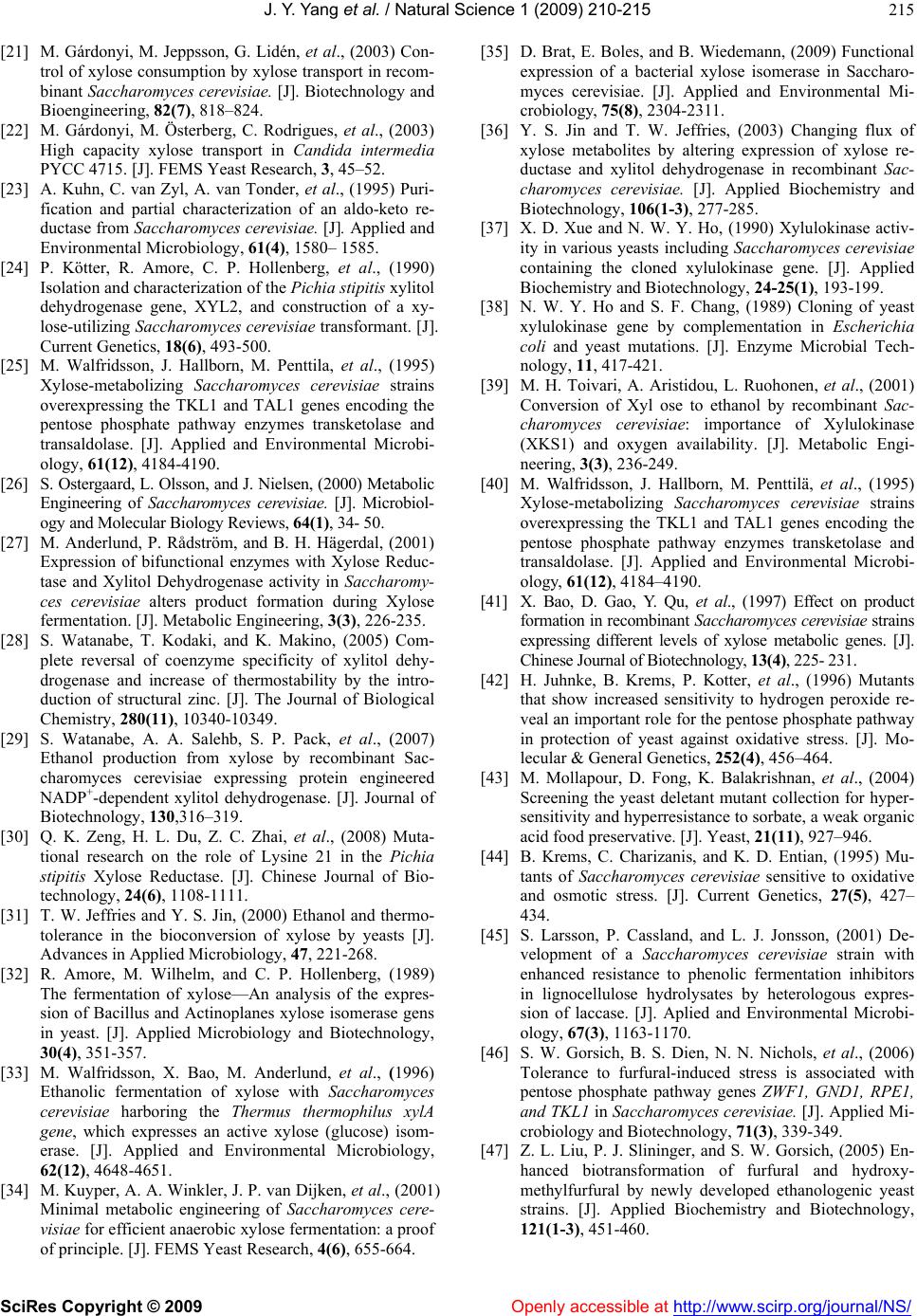
J. Y. Yang et al. / Natural Science 1 (2009) 210-215
SciRes Copyright © 2009 http://www.scirp.org/journal/NS/Openly accessible at
215
[21] M. Gárdonyi, M. Jeppsson, G. Lidén, et al., (2003) Con-
trol of xylose consumption by xylose transport in recom-
binant Saccharomyces cerevisiae. [J]. Biotechnology and
Bioengineering, 82(7), 818–824.
[22] M. Gárdonyi, M. Österberg, C. Rodrigues, et al., (2003)
High capacity xylose transport in Candida intermedia
PYCC 4715. [J]. FEMS Yeast Research, 3, 45–52.
[23] A. Kuhn, C. van Zyl, A. van Tonder, et al., (1995) Puri-
fication and partial characterization of an aldo-keto re-
ductase from Saccharomyces cerevisiae. [J]. Applied and
Environmental Microbiology, 61(4), 1580– 1585.
[24] P. Kötter, R. Amore, C. P. Hollenberg, et al., (1990)
Isolation and characterization of the Pichia stipitis xylitol
dehydrogenase gene, XYL2, and construction of a xy-
lose-utilizing Saccharomyces cerevisiae transformant. [J].
Current Genetics, 18(6), 493-500.
[25] M. Walfridsson, J. Hallborn, M. Penttila, et al., (1995)
Xylose-metabolizing Saccharomyces cerevisiae strains
overexpressing the TKL1 and TAL1 genes encoding the
pentose phosphate pathway enzymes transketolase and
transaldolase. [J]. Applied and Environmental Microbi-
ology, 61(12), 4184-4190.
[26] S. Ostergaard, L. Olsson, and J. Nielsen, (2000) Metabolic
Engineering of Saccharomyces cerevisiae. [J]. Microbiol-
ogy and Molecular Biology Reviews, 64(1), 34- 50.
[27] M. Anderlund, P. Rådström, and B. H. Hägerdal, (2001)
Expression of bifunctional enzymes with Xylose Reduc-
tase and Xylitol Dehydrogenase activity in Saccharomy-
ces cerevisiae alters product formation during Xylose
fermentation. [J]. Metabolic Engineering, 3(3), 226-235.
[28] S. Watanabe, T. Kodaki, and K. Makino, (2005) Com-
plete reversal of coenzyme specificity of xylitol dehy-
drogenase and increase of thermostability by the intro-
duction of structural zinc. [J]. The Journal of Biological
Chemistry, 280(11), 10340-10349.
[29] S. Watanabe, A. A. Salehb, S. P. Pack, et al., (2007)
Ethanol production from xylose by recombinant Sac-
charomyces cerevisiae expressing protein engineered
NADP+-dependent xylitol dehydrogenase. [J]. Journal of
Biotechnology, 130,316–319.
[30] Q. K. Zeng, H. L. Du, Z. C. Zhai, et al., (2008) Muta-
tional research on the role of Lysine 21 in the Pichia
stipitis Xylose Reductase. [J]. Chinese Journal of Bio-
technology, 24(6), 1108-1111.
[31] T. W. Jeffries and Y. S. Jin, (2000) Ethanol and thermo-
tolerance in the bioconversion of xylose by yeasts [J].
Advances in Applied Microbiology, 47, 221-268.
[32] R. Amore, M. Wilhelm, and C. P. Hollenberg, (1989)
The fermentation of xylose—An analysis of the expres-
sion of Bacillus and Actinoplanes xylose isomerase gens
in yeast. [J]. Applied Microbiology and Biotechnology,
30(4), 351-357.
[33] M. Walfridsson, X. Bao, M. Anderlund, et al., (1996)
Ethanolic fermentation of xylose with Saccharomyces
cerevisiae harboring the Thermus thermophilus xylA
gene, which expresses an active xylose (glucose) isom-
erase. [J]. Applied and Environmental Microbiology,
62(12), 4648-4651.
[34] M. Kuyper, A. A. Winkler, J. P. van Dijken, et al., (2001)
Minimal metabolic engineering of Saccharomyces cere-
visiae for efficient anaerobic xylose fermentation: a proof
of principle. [J]. FEMS Yeast Research, 4(6), 655-664.
[35] D. Brat, E. Boles, and B. Wiedemann, (2009) Functional
expression of a bacterial xylose isomerase in Saccharo-
myces cerevisiae. [J]. Applied and Environmental Mi-
crobiology, 75(8), 2304-2311.
[36] Y. S. Jin and T. W. Jeffries, (2003) Changing flux of
xylose metabolites by altering expression of xylose re-
ductase and xylitol dehydrogenase in recombinant Sac-
charomyces cerevisiae. [J]. Applied Biochemistry and
Biotechnology, 106(1-3), 277-285.
[37] X. D. Xue and N. W. Y. Ho, (1990) Xylulokinase activ-
ity in various yeasts including Saccharomyces cerevisiae
containing the cloned xylulokinase gene. [J]. Applied
Biochemistry and Biotechnology, 24-25(1), 193-199.
[38] N. W. Y. Ho and S. F. Chang, (1989) Cloning of yeast
xylulokinase gene by complementation in Escherichia
coli and yeast mutations. [J]. Enzyme Microbial Tech-
nology, 11, 417-421.
[39] M. H. Toivari, A. Aristidou, L. Ruohonen, et al., (2001)
Conversion of Xyl ose to ethanol by recombinant Sac-
charomyces cerevisiae: importance of Xylulokinase
(XKS1) and oxygen availability. [J]. Metabolic Engi-
neering, 3(3), 236-249.
[40] M. Walfridsson, J. Hallborn, M. Penttilä, et al., (1995)
Xylose-metabolizing Saccharomyces cerevisiae strains
overexpressing the TKL1 and TAL1 genes encoding the
pentose phosphate pathway enzymes transketolase and
transaldolase. [J]. Applied and Environmental Microbi-
ology, 61(12), 4184–4190.
[41] X. Bao, D. Gao, Y. Qu, et al., (1997) Effect on product
formation in recombinant Saccharomyces cerevisiae strains
expressing different levels of xylose metabolic genes. [J].
Chinese Journal of Biotechnology, 13(4), 225- 231.
[42] H. Juhnke, B. Krems, P. Kotter, et al., (1996) Mutants
that show increased sensitivity to hydrogen peroxide re-
veal an important role for the pentose phosphate pathway
in protection of yeast against oxidative stress. [J]. Mo-
lecular & General Genetics, 252(4), 456–464.
[43] M. Mollapour, D. Fong, K. Balakrishnan, et al., (2004)
Screening the yeast deletant mutant collection for hyper-
sensitivity and hyperresistance to sorbate, a weak organic
acid food preservative. [J]. Yeast, 21(11), 927–946.
[44] B. Krems, C. Charizanis, and K. D. Entian, (1995) Mu-
tants of Saccharomyces cerevisiae sensitive to oxidative
and osmotic stress. [J]. Current Genetics, 27(5), 427–
434.
[45] S. Larsson, P. Cassland, and L. J. Jonsson, (2001) De-
velopment of a Saccharomyces cerevisiae strain with
enhanced resistance to phenolic fermentation inhibitors
in lignocellulose hydrolysates by heterologous expres-
sion of laccase. [J]. Aplied and Environmental Microbi-
ology, 67(3), 1163-1170.
[46] S. W. Gorsich, B. S. Dien, N. N. Nichols, et al., (2006)
Tolerance to furfural-induced stress is associated with
pentose phosphate pathway genes ZWF1, GND1, RPE1,
and TKL1 in Saccharomyces cerevisiae. [J]. Applied Mi-
crobiology and Biotechnology, 71(3), 339-349.
[47] Z. L. Liu, P. J. Slininger, and S. W. Gorsich, (2005) En-
hanced biotransformation of furfural and hydroxy-
methylfurfural by newly developed ethanologenic yeast
strains. [J]. Applied Biochemistry and Biotechnology,
121(1-3), 451-460.